Abstract
While uniparental transmission of mtDNA is widespread and dominating in eukaryotes leaving mutation as the major source of genotypic diversity, recently, biparental inheritance of mitochondrial genes has been demonstrated in reciprocal crosses of Pelargonium zonale and P. inquinans. The thereby arising heteroplasmy carries the potential for recombination between mtDNAs of different descent, i.e. between the parental mitochondrial genomes. We have analyzed these Pelargonium hybrids for mitochondrial intergenomic recombination events by examining differences in DNA blot hybridization patterns of the mitochondrial genes atp1 and cob. Further investigation of these genes and their flanking regions using nucleotide sequence polymorphisms and PCR revealed DNA segments in the progeny, which contained both P. zonale and P. inquinans sequences suggesting an intergenomic recombination in hybrids of Pelargonium. This turns Pelargonium into an interesting subject for studies of recombination and evolutionary dynamics of mitochondrial genomes.
Similar content being viewed by others
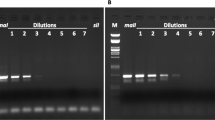
Avoid common mistakes on your manuscript.
Introduction
In the great majority of plant species, mitochondrial (mt) genes are inherited maternally (reviewed in Mogensen 1996; Hagemann 2004; Barr et al. 2005). While the parental nuclear genomes normally undergo extensive recombination between the paternal and maternal genomes in the progeny of sexual crosses, uniparental transmission of organellar DNAs prevents intergenomic recombination. However, species which are regarded as strictly maternal in their mitochondrial inheritance often exhibit a rare contribution of paternal chondromes (mt genomes) to the progeny leading to heteroplasmy (presence of organellar genomes differing in their sequence), which is required for intergenomic recombination (Wagner et al. 1991; Bentley et al. 2010). Heteroplasmy may arise also from spontaneous mutations in mtDNA, which are rather frequent in mammalia, may cause diseases, are discussed to contribute to the aging of organisms including man, and have recently been shown to be the basis for the generation of new combinations of mutant loci by intermolecular, intragenomic recombination in mouse cell lines (Fan et al. 2012). Plant mtDNAs exhibit a remarkably low rate of spontaneous mutations. However, there is another source of heteroplasmy in plants, the generation of new subgenomic mtDNA molecules through homologous recombination between repeats within the organellar genome. The plant chondromes are characterized by high levels of this intragenomic recombination. A number of enzymes involved in recombination have been identified and found to be encoded in the nuclear genome. As a consequence, these nuclear gene products have to be imported into the organelle making the recombinational activity of mtDNA dependent on nuclear control mechanisms (Arrieta-Montiel et al. 2009; reviewed in Maréchal and Brisson 2010; Woloszynska 2010; Arrieta-Montiel and Mackenzie 2011).
In addition to heteroplasmy, fusion of mitochondria is discussed as a necessary precondition for the physical contact of mtDNA of different descent and consequently for intergenomic recombination (Lackner and Nunnari 2009; Takano et al. 2010). It is well known that plant mitochondria are highly dynamic organelles undergoing continuous fusion and fission (Arimura et al. 2004; Sheahan et al. 2005; reviewed in Logan 2010).
Fungi exhibit intergenomic recombination of mtDNA under conditions in which heteroplasmy is forced (Rowlands and Turner 1975; Takano et al. 2010). In the basidiomycete fungus Armillaria gallica recombination between mtDNAs of different descent has been proven for the first time in a natural population (Saville et al. 1998). Intergenomic recombination of mt genomes in plants has been reported predominantly for cytoplasmatic hybrids (cybrids) originating from artificially induced fusion of protoplasts (Belliard et al. 1979; Rothenberg and Hanson 1987; Akagi et al. 1995). There are only a few examples for the exchange between plant mt genomes of different descent in vivo (Städler and Delph 2002; Jaramillo-Correa and Bousquet 2005). Plant chondromes are characterized by the existence of numerous sublimons, i.e. substoichiometric subgenomic DNA molecules that may not be detectable by convential techniques (Small et al. 1989). The level of sublimons may, however, drastically raise due to changes in the nuclear genome like mutations or appearance of new alleles after crossing or somatic hybridization. This phenomenon is called substoichiometric shifting and has to be taken into account in studies on intergenomic recombination of mtDNA in plants (Maréchal and Brisson 2010; Woloszynska 2010; Arrieta-Montiel and Mackenzie 2011).
Pelargonium species are the only higher plants for which a nearly equal transmission of maternal and paternal mitochondria to the progeny has been demonstrated (Weihe et al. 2009). The biparental transmission leads to pronounced heteroplasmy in hybrid plants. Although biparental inheritance of organellar genes is a prerequisite for intergenomic recombination in the progeny of sexual crosses, there are cases of biparental transmission without recombination of the organellar genomes from the two parents (Birky 1995). Here we report on the intergenomic recombination of mtDNAs in Pelargonium hybrids. Using sequence polymorphism, PCR fragment analyses and DNA blot hybridization, we demonstrate recombination between mt genomes in hybrids of P. zonale and P. inquinans.
Materials and methods
Plant material
We studied the leaves of Pelargonium zonale (L.) L’Her., P. inquinans (L.) L’Her., and of the hybrids P. zonale × P. inquinans, and P. inquinans × P. zonale. The hybrids show variegation due to bleaching of the P. inquinans but not of the P. zonale chloroplasts most likely because of the incompatibility of the former with the hybrid nuclear genome (Weihe et al. 2009). The original crosses were made with one plant of each species in 1984. The cross P. zonale × P. inquinans resulted in 5 bleached, 2 variegated and 23 green seedlings, the cross P. inquinans × P. zonale in 3 bleached, 8 variegated and 2 green seedlings (Pohlheim 1986). The two parental and the variegated hybrid plants have been maintained in a greenhouse. We used the plant material derived from cuttings made from branches of the parental plants and two variegated hybrid plants (P. inquinans × P. zonale–i × z I, and P. zonale × P. inquinans–z × i IV; Weihe et al. 2009) in 2007. During the present study, the plants were kept under long day (16 h light/8 h darkness) conditions.
DNA extraction
Total DNA was isolated from leaves using the protocol of Khanuja (1999).
DNA blot hybridization
5 μg of genomic DNA extracted from fresh leaf tissue of P. zonale, P. inquinans and their hybrids originating from reciprocal crosses was digested with the restriction enzymes HindIII or TaiI, respectively, electrophoretically separated on 0.8 % agarose gels, and transferred to positively charged nylon membranes by standard capillary blotting. Following a wash step with 2× SSC, membranes were hybridized at 65 °C in 7 % SDS, 250 mM sodium phosphate, pH 7.2. After DNA hybridization, the filters were washed at 65 °C in buffers with decreasing SDS and SSC concentrations down to 0.1 % SDS, 0.1 % SSC. Hybridization probes (PCR fragments, amplified from the mt genes atp1 and cob, see below) were generated by 32P-labeling using random oligonucleotide primers according to standard techniques.
Amplification of gene-flanking regions using inverse PCR
1 μg of genomic DNA was digested overnight with restriction enzymes HindIII or TaiI, respectively. Residual enzyme activity was removed by incubation at 65 °C for 30 min and subsequent ethanol precipitation. The digested DNA was self-ligated at room temperature overnight in a total volume of 400 μl in the presence of 45 units of T4 DNA ligase. The DNA samples were extracted with phenol/chloroform (1:1, v/v) to remove the T4 ligase and subsequently precipitated with ethanol. 50 ng of religated genomic DNA was used for the following PCR employing primers annealing to regions of the studied mitochondrial genes atp1 and cob and directed into the gene-flanking regions of unknown sequence. For primers used in the present study, see Supplementary Materials, Table S1—List of oligonucleotide primers. The PCRs were performed in a total volume of 50 μl under the same conditions as described for amplification of mitochondrial gene fragments (see below).
Amplification of mitochondrial gene fragments and sequencing
GenBank entries of the mitochondrial genes atp1 and cob of Pelargonium × hortorum (accession numbers DQ317063 [atp1], DQ317065 [cob]) were used to design gene-specific primers. The PCRs were performed in a total volume of 50 μl containing 5 μl 10× reaction buffer, 1 μl 10 mM dNTP, 10 pmol of forward and reverse primer, 1 μl of template DNA (50 ng for inverse PCR), and 2.5 units Taq DNA polymerase. Reactions were incubated for 1 min at 95 °C, and then run for 40 cycles (30 cycles for inverse PCR) at 94 °C for 30 s, 56–63 °C (depending on the annealing temperature of the primers) for 30 s, and 72 °C for 60 s, followed by a final extension at 72 °C for 7 min. Depending on their size, the amplicons were separated electrophoretically on 0.8–1.5 % agarose gels, the fragments were cut out from the gel under UV light, and the DNA was purified using Qiagen spin columns according to the instructions of the manufacturer. The fragments were sequenced directly using amplification primers and BigDye terminator chemistry (Applied Biosystems). DNA sequences were aligned using the software DNAStar Lasergene v7.1.
Results and Discussion
DNA blot hybridization suggests recombination between mt genomes
To monitor mitochondrial intergenomic recombination, DNA blot analyses were performed with mitochondrial atp1 and cob coding sequences as probes showing a different hybridization pattern between the two parental species P. zonale and P. inquinans (Fig. 1). Due to the biparental mode of inheritance of mitochondria (Weihe et al. 2009), the presence of both of these patterns can be expected to occur in hybrid plants. In case of the material examined in the present study, P. inquinans × P. zonale (hybrid i × z, clone I) and P. zonale × P. inquinans (hybrid z × i, clone IV), we observed mainly paternal mtDNA polymorphisms in a previous investigation (Weihe et al. 2009). While we indeed found the paternal patterns in z × i IV, novel hybridization patterns were detected in the hybrid i x z I suggesting recombination events in these genome areas between the mtDNA of the two parents (Fig. 1).
Southern hybridization of genomic DNA from plant leaves of P. zonale, P. inquinans and hybrid plants with atp1 and cob gene probes (see Figs. 2, 3 for exact localization of the probes). a atp1, DNA digested with HindIII. b cob, DNA digested with TaiI. Note the occurrence of novel hybridizing fragments in the hybrid plant i × z I compared to P. zonale and P. inquinans parental fragments. No novel gene fragments were detected in the hybrid i × z IV. Exact sizes of the fragments are given as determined by inverse PCR and sequencing of the fragments
The hybridization pattern of the mt gene atp1 differs between P. zonale and P. inquinans displaying each one band of ca. 3.0 kb (P. zonale) and ca. 2.1 kb (P. inquinans) (see Fig. 1a). As the hybridization probe covers almost the full length of the coding region, it can be concluded that the Pelargonium mtDNA contains only one functional, complete copy of atp1. From the two hybrids studied, one (z × i IV) shows a band exactly of the same size as that in P. inquinans which can be explained by complete sorting-out of the maternal P. zonale mtDNA (at least the fragment detected by the hybridization probe) in this plant, as a consequence of the proposed random segregation of mitochondria and their DNAs during plant development observed in Pelargonium (Weihe et al. 2009). In hybrid i × z I, however, a novel hybridizing fragment of approximately 3.3 kb was observed although we previously detected exclusively the paternal mtDNA polymorphisms in this material.
DNA blots performed with the mt gene cob reveal a hybridization pattern with one band showing up in each of the parents (ca. 2.6 kb in P. zonale and ca. 2.0 kb in P. inquinans, Fig. 1b). One of the hybrids (z × i IV) shows a band of the same size as that of P. inquinans, again demonstrating segregation of maternal and paternal mtDNAs in the hybrid leading in this case to leaves containing the detected paternal mtDNA. The other hybrid studied, i × z I, exhibits a hybridization fragment of ca. 2.7 kb, clearly different from the maternal fragment and slightly larger in size than that from the paternal plant, again suggesting this hybrid to bear recombined mtDNA.
Sequence analysis of the two mt loci
The novel hybridizing fragments observed in the DNA blots of the mitochondrial genes atp1 and cob in one of the two hybrid plants indicate the possible recombination events at these genomic loci and are of peculiar interest for further investigation. Therefore, the fragments comprising the mitochondrial genes atp1 and cob, respectively, and their flanking regions were amplified using inverse PCR. The products of the inverse PCR were sequenced and assembled with the coding regions of the genes obtained by conventional PCR (Figs. 2, 3). The sequences are available at EMBL under accession numbers HE984330, HE984331 and HE984332 (atp1 locus) and HE984327, HE984328 and HE984329 (cob locus). For an alignment, see Supplementary Materials, Figs. S1 and S2.
Schematic alignment of the sequenced atp1 locus. The atp1 open reading frame is indicated by its start (triangle) and stop (circle) codons. The observed nucleotide polymorphisms between P. inquinans and P. zonale (Weihe et al. 2009) in the atp1 coding sequence are highlighted (TGA vs. AAC, GA vs. TC). Differences in the sequences of the flanking regions are indicated by different grades of shading (white/grey/black). The position of the probe used in DNA blot hybridization is indicated. Specific parts of the hybrid fragment were examined for their existence in P. zonale or P. inquinans using PCR. Numbered fragments indicated by solid lines were also detected in P. inquinans, those indicated by dashed lines also in P. zonale, and those indicated by dotted lines in neither P. inquinans nor P. zonale, but exclusively in the hybrid. The asterisks indicate the possible recombination sites deduced from the PCR results
Schematic alignment of the sequenced cob locus. The cob open reading frame is indicated by its start (triangle) and stop codons (circle). In P. inquinans, no stop codon was detected. Differences in the sequences of the flanking regions are indicated by different grades of shading (white/grey/black). Nucleotide polymorphisms (GA vs. TC and A vs. C) are highlighted. The position of the probe used in DNA blot hybridization is indicated. Specific parts of the hybrid fragment were examined for their existence in P. zonale or P. inquinans using PCR. Numbered fragments indicated by solid lines were also detected in P. inquinans, those indicated by dashed lines also in P. zonale, and those by dotted lines in neither P. inquinans nor P. zonale, but exclusively in the hybrid. The asterisk indicates the possible recombination site deduced from the PCR results
One short nucleotide polymorphism between P. zonale and P. inquinans (TGA vs. AAC) was observed in the atp1 coding sequence, a further nucleotide exchange at the position of the stop codon (taGA vs. taTC, stop codon underlined) leads to a 78 nt longer open reading frame in P. inquinans. The sequence of the ORF of the hybrid (i × z I) is identical with that of P. zonale, which is in accordance with our previous finding of the paternal atp1 polymorphisms in this hybrid (Weihe et al 2009). The flanking regions of all three examined fragments differ from each other as indicated by their shading in Fig. 2.
The cob coding sequence of P. zonale and P. inquinans differ by the previously identified nucleotide polymorphisms (Weihe et al. 2009). Based on alignments of the sequence with cob genes from other plant species (not shown) we propose the indicated CAA (Fig. 3) to serve as a stop codon, which is presumably edited in the mRNA to UAA. Although it has been reported that there is no or only little RNA editing in mitochondria of Geraniceae (Barr et al. 2005), the sequence alignment data strongly suggest that the CAA codon found at the 3′ end of the cob gene of P. zonale is indeed an editing site. No putative stop codon or editable codon to serve as such was detected in P. inquinans. A potential (editable) in-frame stop codon occurs only far downstream and would lead to a considerably longer polypeptide (data not shown). Thus, most likely, the P. inquinans cob gene contains an intron in its 3′ part. The cob coding sequence and the 3′ flanking region of the hybrid i × z I are identical with the respective P. zonale sequences (see Fig. 3), whereas the upstream region of the gene differs in all three cases, i.e. the 5′ sequence of the hybrid i × z I shares no similarity with those of the parental plants.
Origin of the i × z I hybrid fragments
Sequence analysis indicated that the open reading frames of atp1 and cob of the i × z I hybrid fragments originate from P. zonale. In the case of an intergenomic recombination, P. inquinans-specific sequences should be detected alongside the P. zonale-specific ones. A series of PCR experiments was designed to determine the origin of the hybrid fragments. In all cases, the fragments obtained by PCR were of exactly the size as predicted from localization of the primers on the hybrid sequence (see Figs. 2, 3 for localization and sizes of the fragments), and in none of the amplification reactions were additional fragments detected. The most upstream flanking region of the hybrid atp1 could only be detected in P. inquinans (see Fig. 2, fragment #1), whereas sequences closer to the ORF were amplified only from P. zonale (fragment #2, note the position of its 5′ end in a sequence region different beween the hybrid i × z I and P. inquinans). The P. inquinans-specific flanking region may not necessarily originate from a putative second (incomplete) atp1 locus. A PCR covering the region from the most upstream flanking region and the ORF did not yield any amplicon in neither P. zonale nor P. inquinans (fragment #3) which could only be explained by the occurrence of a novel fragment through recombination in the hybrid, consisting of both P. zonale- and P. inquinans-specific sequences. Fragment #6, covering the 3′ flanking region and a larger part of the 3′ atp1 coding sequence could not be amplified from the parental plants either. To increase the sensitivity of detection, the PCR products of the hybrid-specific fragments #3 and #6, amplified from both parental plants and the hybrid, were blotted and hybridized with the radioactively labeled fragments obtained from the hybrid plant (see Fig. 4). This technique is recommended to detect sublimons occurring at very low substoichiometric levels (Janska et al. 1998; Woloszynska et al. 2001). In neither of the parents signals indicating substoichiometric amounts of the fragments were detected. Thus, the absence of the hybrid-specific 5′ and 3′ atp1 fragments in P. inquinans and P. zonale, most likely, excludes sublimons and their substoichiometric shifting as an explanation for the appearance of the novel fragment in the hybrid plant.
Hybrid-specific PCR fragments are not detected in the parental plants. a Amplification of fragments #3 and #6 (see Fig. 2) from the hybrid plant i × z I, and the parental plants P. inquinans and P. zonale. b Hybridization of the blotted PCR samples with the hybrid-specific fragment as probe. 1 μl of the reaction volume of the hybrid-specific PCR was run alongside total volumes (50 μl) from P. inquinans and P. zonale PCRs
More distant parts of the atp1 downstream flanking region (fragments #4 and #5) were detected in P. inquinans. As this region differs in its composition from the atp1-P. inquinans fragment detected in the DNA blot (Fig. 1a), one has to assume the existence of more than one copy of atp1 that might have evolved through intragenomic recombination. This assumption was confirmed by another DNA blot analysis. A part of the downstream flanking region of the hybrid that is also detectable in P. inquinans (fragment #5) was used as probe for hybridization (Fig. 5a). The two additional bands showing up in the blot (marked by asterisks) imply additional copies of atp1 sequences in the mt genome of P. inquinans. These gene copies are assumed to be incomplete as DNA hybridization with a probe spanning the nearly complete ORF of atp1 revealed only one band for P. inquinans (see Fig. 1a). A contiguous sequence between fragments #1 and #5 could not be amplified from P. inquinans or P. zonale (data not shown), an indication for different origins of the two flanking regions of the atp1 locus of the hybrid fragment.
DNA blot hybridization reveals additional atp1 (a) and cob (b) gene copies. Amplicons of flanking regions of the hybrid i × z I also detected in P. inquinans (fragment #5 for atp1, see Fig. 2; fragment #8 for cob, see Fig. 3) were used as hybridization probes. DNA was digested with HindIII (a) and TaiI (b), respectively. Asterisks mark bands which imply the existence of additional (incomplete) copies of atp1 and cob gene sequences in P. inquinans
The cob upstream flanking region of the hybrid could be amplified only from P. inquinans, but not from P. zonale (Fig. 3, fragments #7 and #8). As this region differs in its composition from the cob-P. inquinans fragment detected in the DNA blot (Fig. 1b), it should originate from another cob gene copy in the mt genome of P. inquinans. Again, DNA hybridization with the flanking region of the hybrid (fragment #8) as probe confirmed the existence of several (partial) cob gene copies, since two additional bands were detected in P. inquinans (Fig. 5b). As for atp1, the additional gene copies in the mt genome of P. inquinans have to be assumed to be incomplete as hybridization with a probe spanning the nearly complete ORF of cob resulted in only one band (see Fig. 1b). Amplicons including a slightly longer stretch of the 5′ cob coding region (fragment #9) were not detected in either of the parents, indicating the occurrence of a novel fragment in the hybrid. Furthermore, cob downstream flanking regions (fragment #10) were detected in P. zonale leaving intergenomic recombination as the best explanation for the appearance of the novel fragment.
In the present study, two randomly chosen mitochondrial loci, atp1 and cob, were analyzed, and for both of them we were able to demonstrate intergenomic recombination in one Pelargonium hybrid plant, i × z I. One could expect that extended analysis of the the whole mitochondrial genome of Pelargonium hybrids will reveal numerous more recombination events, probably also in the hybrid plant z × i IV, which did not exhibit recombination at the two loci studied here. As a consequence of biparental inheritance in Pelargonium, “pure” maternal or paternal mt genomes seem to be replaced by hybrid chondromes, generated through intergenomic recombination.
The Pelargonium family of Geraniceae has been reported to exhibit an unusual high rate of synonymous substitutions in the mtDNA (Parkinson et al. 2005). In addition to high mutation rates, intergenomic recombination might as well have contributed to accelerated evolution of the mitochodrial genome of Pelargonium.
Abbreviations
- mt:
-
Mitochondrial
References
Akagi H, Shimada H, Fujimura T (1995) High-frequency inter-parental recombination between mitochondrial genomes of rice cybrids. Curr Genet 29:58–65
Arimura S, Yamamoto J, Aida GP, Nakazono M, Tsutsumi N (2004) Frequent fusion and fission of plant mitochondria with unequal nucleoid distribution. Proc Natl Acad Sci USA 101:7805–7808
Arrieta-Montiel M, Mackenzie S (2011) Plant mitochondrial genomes and recombination. In: Kempken F (ed) Plant mitochondria, advances in plant biology, 1st edn. Springer, New York, pp 65–82
Arrieta-Montiel MP, Shedge V, Davila J, Christensen AC, Mackenzie SA (2009) Diversity of the Arabidopsis mitochondrial genome occurs via nuclear-controlled recombination activity. Genetics 183:1261–1268
Barr CM, Neiman M, Taylor DR (2005) Inheritance and recombination of mitochondrial genomes in plants, fungi and animals. New Phytol 168:39–50
Belliard G, Vedel F, Pelletier G (1979) Mitochondrial recombination in cytoplasmic hybrids of Nicotiana tabacum by protoplast fusion. Nature 281:401–403
Bentley KE, Mandel JR, McCauley DE (2010) Paternal leakage and heteroplasmy of mitochondrial genomes in Silene vulgaris: evidence from experimental crosses. Genetics 185:961–968
Birky CW (1995) Uniparental inheritance of mitochondrial and chloroplast genes: mechanisms and evolution. Proc Natl Acad Sci USA 92:11331–11338
Fan W, Lin CS, Potluri P, Procaccio V, Wallace DC (2012) mtDNA lineage analysis of mouse L-cell lines reveals the accumulation of multiple mtDNA mutants and intermolecular recombination. Genes Develop 26:384–394
Hagemann R (2004) The sexual inheritance of plant organelles. In: Daniel H, Chase C (eds) Molecular biology and biotechnology of plant organelles, 1st edn. Springer, Dordrecht, pp 93–113
Janska H, Sarria R, Woloszynska M, Arrieta-Montiel M, Mackenzie SA (1998) Stoichiometric shifts in the common bean mitochondrial genome leading to male sterility and spontaneous reversion to fertility. Plant Cell 10:1163–1180
Jaramillo-Correa JP, Bousquet J (2005) Mitochondrial genome recombination in the zone of contact between two hybridizing conifers. Genetics 171:1951–1962
Khanuja SPS, Shasany AK, Darokar MP, Kumar S (1999) Rapid isolation of DNA from dry and fresh samples of plants producing large amounts of secondary metabolites and essential oils. Plant Mol Biol Report 17:1–7
Lackner LL, Nunnari JM (2009) The molecular mechanism and cellular functions of mitochondrial division. BBA-Mol Basis Dis 1792:1138–1144
Logan DC (2010) Mitochondrial fusion, division and positioning in plants. Biochem Soc Trans 38:789–795
Maréchal A, Brisson N (2010) Recombination and the maintenance of plant organelle genome stability. New Phytol 186:299–317
Mogensen HL (1996) The hows and whys of cytoplasmic inheritance in seed plants. Am J Bot 83:383–404
Parkinson CL, Mower JP, Qiu YL, Shirk AJ, Song K, Young ND, DePamphilis CW, Parker JD (2005) Multiple major increases and decreases in mitochondrial substitution ratesin the plant family Geraniceae. BMC Evol Biol 5:73
Pohlheim F (1986) Hybrid variegation in crosses between Pelargonium zonale (L.) l`Herit. Ex Ait. and Pelargonium inquinans (L.) l`Herit. Ex Ait Plant Breed 97:93–96
Rothenberg M, Hanson MR (1987) Recombination between parental mitochondrial DNA following protoplast fusion can occur in a region which normally does not undergo intragenomic recombination in parental plants. Curr Genet 12:235–240
Rowlands RT, Turner G (1975) Three-marker extranuclear mitochondrial crosses in Aspergillus nidulans. Mol Genet Genomics 141:69–79
Saville BJ, Kohli Y, Anderson JB (1998) mtDNA recombination in a natural population. Proc Natl Acad Sci USA 95:1331–1335
Sheahan MB, McCurdy DW, Rose RJ (2005) Mitochondria as a connected population: ensuring continuity of the mitochondrial genome during plant cell dedifferentiation through massive mitochondrial fusion. Plant J 44:744–755
Small I, Suffolk R, Leaver CJ (1989) Evolution of plant mitochondrial genomes via substoichiometric intermediates. Cell 58:69–76
Städler T, Delph LF (2002) Ancient mitochondrial haplotypes and evidence for intragenic recombination in a gynodioecious plant. Proc Natl Acad Sci USA 99:11730–11735
Takano H, Onoue K, Kawano S (2010) Mitochondrial fusion and inheritance of the mitochondrial genome. J Plant Res 123:131–138
Wagner DB, Dong J, Carlson MR, Yanchu AD (1991) Paternal leakage of mitochondrial DNA in Pinus. Theor Appl Genet 82:510–514
Weihe A, Apitz J, Salinas A, Pohlheim F, Börner T (2009) Biparental inheritance of plastidial and mitochondrial DNA and hybrid variegation in Pelargonium. Mol Genet Genomics 282:587–593
Woloszynska M (2010) Heteroplasmy and stoichiometric complexity of plant mitochondrial genomes—though this be madness, yet there’s method in’t. J Exp Bot 61:657–671
Woloszynska M, Kieleczawa J, Ornatowska M, Wozniak M, Janska H (2001) The origin and maintenance of the small repeat in the bean mitochondrial genome. Mol Genet Genomics 265:865–872
Acknowledgments
The excellent technical assistance of Cornelia Stock is gratefully acknowledged.
Author information
Authors and Affiliations
Corresponding author
Additional information
A contribution to the Special Issue on Evolution and Biogenesis of Chloroplasts and Mitochondria.
Electronic supplementary material
Below is the link to the electronic supplementary material.
Rights and permissions
About this article
Cite this article
Apitz, J., Weihe, A., Pohlheim, F. et al. Biparental inheritance of organelles in Pelargonium: evidence for intergenomic recombination of mitochondrial DNA. Planta 237, 509–515 (2013). https://doi.org/10.1007/s00425-012-1768-x
Received:
Accepted:
Published:
Issue Date:
DOI: https://doi.org/10.1007/s00425-012-1768-x