Abstract
We have previously shown that post-exercise inspiratory resistive loading (IRL) reduces blood lactate ([Lac −b ]). In this study, we tested the hypothesis that IRL during recovery could improve subsequent exercise performance. Eight healthy men underwent, on different days, two sequential 30-s, cycle ergometer Wingate tests. During the 10-min recovery period from test 1, subjects breathed freely or through an inspiratory resistance (15 cm H2O) with passive leg recovery. Arterialized [Lac −b ] values, perceptual scores (Borg), cardiac output by impedance cardiography (QT), and changes in the deoxygenation status of the M. vastus lateralis by near-infrared spectroscopy (ΔHHb), were recorded. [Lac −b ] was significantly reduced after 4 min of recovery with IRL (peak [Lac −b ] 12.5 ± 2.3 mmol l−1 with free-breathing vs. 9.8 ± 1.5 mmol l−1 with IRL). Effort perception was reduced during late recovery with IRL compared with free-breathing. Cardiac work was increased with IRL, since heart rate and QT were elevated during late recovery. Peripheral muscle reoxygenation, however, was significantly impaired with IRL, suggesting that post-exercise convective O2 delivery to the lower limbs was reduced. Importantly, IRL had a dual effect on subsequent performance, i.e., improvement in peak and mean power, but increased fatigue index (P < 0.05). Our data demonstrate that IRL after a Wingate test reduces post-exercise effort perception and improves peak power on subsequent all-out maximal-intensity exercise.
Similar content being viewed by others
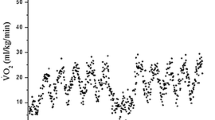
Avoid common mistakes on your manuscript.
Introduction
Muscle and blood lactic acid accumulation have long been related to impaired exercise performance (Hill et al. 1924) and several strategies have been used to alleviate the physiological and subjective burdens associated with increased lactic acidemia (Chiappa et al. 2008b). The subject is of special concern when the lactic–glycolytic metabolic pathway is heavily requested for ATP regeneration, such as during short-term maximal-intensity exercise, e.g., the 30-s, all-out Wingate test (Dotan 2006). In this context, the respiratory (diaphragm and accessory) muscles are particularly suited to act as lactate (Lac−) consumers after exercise. In fact, these muscles may have an even higher potential for post-exercise Lac− removal than the appendicular muscles as they present with increased oxidative potential, higher proportion of type I fibers, and enhanced post-exercise metabolic activity (Fregosi and Dempsey 1986). Moreover, the diaphragm is profusely perfused during exercise and early recovery, presenting with well-developed mechanisms of blood flow preservation (Guenette et al. 2008). Therefore, it is conceivable that increased respiratory muscle work with inspiratory resistive loading (IRL) could accelerate post-Wingate blood lactate (Lac −b ) removal. Indeed, we have recently shown that post-exercise Lac− clearance was significantly increased by adding IRL to passive leg recovery from maximal incremental exercise test in healthy individuals (Chiappa et al. 2008b). In that specific study, however, we did not address whether this strategy would actually be able to improve performance on subsequent exercise. This is particularly important because IRL might also have deleterious effects on peripheral muscle perfusion due a metaboreflex-mediated blood flow “stealing” effect, i.e., blood flow redistribution from the legs to respiratory muscles secondary to fatiguing contractions of the diaphragm (Borghi-Silva et al. 2008; Chiappa et al. 2008c; Harms et al. 1997; Sheel et al. 2001).
This study, therefore, was performed to investigate the physiological and perceptual effects of 10-min, post-Wingate IRL combined with passive leg recovery in a group of young healthy males. We hypothesized that: (i) post-Wingate IRL would be associated with lower [Lac −b ] levels and diminished sense of leg fatigability compared to free-breathing recovery and (ii) these effects would positively impact upon performance on a subsequent Wingate test.
Methods
Subjects
Eight healthy males (mean ± SD age = 29.0 ± 3.4 years; height = 172 ± 7 cm, and body mass = 78 ± 8 kg) volunteered for study participation. The subjects had a maximal oxygen uptake of 3.74 ± 0.50 l min−1 or 48 ± 1 ml kg−1 min−1 (range: 40–55 ml kg−1 min−1) assessed on a previous ramp-incremental cycle ergometer exercise test. Subjects were free from cardiovascular, respiratory, osteomuscular or metabolic diseases. All participants underwent a comprehensive pre-test clinical evaluation, including spirometry as well as resting and exercise electrocardiograms. None of the subjects took any medication and they did not smoke. All subjects abstained from heavy exercise and caffeine prior to testing. Although subjects were physically active, they were not engaged in a specific training regimen. None of the subjects had participated in our previous study with IRL (Chiappa et al. 2008b). Subjects were informed of all the discomforts and risks involved, and their written consent was obtained before the study. The experimental protocol was approved by the Medical Ethics Committee of the Federal University of São Paulo.
Protocol
Subjects came to the laboratory on two different days, at the same time of the day, with a 48 h interval. During each day they performed two Wingate tests separated by a 15 min recovery period in which subjects breathed without resistance or through an inspiratory resistance during the first 10 min. The order of the tests with or without resistance was randomly assigned. Subjects breathed through a Lloyd valve (Warren E. Collins, Braintree, MA) and a threshold inspiratory muscle training device (Threshold Inspiratory Muscle Trainer, Healthscan Products, Cedar Grove, New Jersey, USA) was inserted in the inspiratory part of the valve. The device was used without resistance or with an inspiratory resistance set at 15 cmH2O. We have previously shown that the addition of this resistance reduces [Lac –b ] during recovery (Chiappa et al. 2008b). Five minutes after the end of this period, the subjects underwent the second Wingate test. Cardiac output (impedance cardiography), changes in the deoxygenation status of the M. vastus lateralis by near-infrared spectroscopy, arterialized [Lac −b ] values, blood arterial pressure response, and perceptual scores (Borg) were obtained before tests, at the peak of the Wingate tests, and every 2 min during recovery the tests.
Wingate exercise tests
Subjects were required to have their last meal at least 3 h before the test, to avoid beverages containing alcohol or caffeine, and they were told to consume the same meals and drink the same water volume before each test. All subjects were fully familiarized with laboratory testing, having previously performed graded and sprint tests on a cycle ergometer. The 30-s Wingate tests consisted of pedaling as fast as possible against a braking force corresponding to 10% of the body weight on a cycle ergometer (The Bike™, Cybex, Division of Lumex, Ronkonkoma, NY, USA) (Dotan 2006). Mean power was computed by the ergometer every 5 s, accounting for the load on the flywheel and crank kinematics. From these data, we obtained the area under the power curve by trapezoid integration (AUCPW in arbitrary units; SigmaPlot 10.0, Systat Software, San Jose, Ca, USA). Peak power was defined as the highest value achieved at any given 5-s bin period and the fatigue index was calculated by the following equation: (peak power – minimal power) × 100/peak power (Dotan 2006).
Blood lactate
Arterialized capillary blood (100 μl) was sampled from a hyperemic earlobe at rest, immediately after the Wingate tests (bout 1 and 2), and serially during the recovery period (2nd, 4th, 6th, 8th, 10th and 15th min) of the first bout. The samples were deproteinized and analyzed by a validated system (Yellow Springs 2.700 STAT Plus, Yellow Springs Instruments, OH, USA). The area under the [Lac −b ] curve in the recovery period was also calculated (AUCLAC in arbitrary units; SigmaPlot 10.0, Systat Software, San Jose, Ca, USA).
Perceptual responses
Subjects were inquired about the perception of “leg effort” and “breathlessness” by using the 0–10 category-ratio Borg scale (Hamilton et al. 1995) at exercise cessation and serially during the recovery period (2nd, 4th, 6th, 8th, 10th and 15th min) of the first Wingate test.
Central hemodynamics
Cardiac output (Q T) and stroke volume (SV) were measured non-invasively before tests, at the peak of the Wingate tests, and every 2 min during recovery the tests by using impedance cardiography (PhysioFlow PF-05™, Manatec Biomedical, France) as previously described (Charloux et al. 2000; Chiappa et al. 2008a). Before each exercise test, the system was auto-calibrated taking into consideration age, stature, body mass, and blood pressure values. In previous experiments, we have shown that the reproducibility of changes in Q T and SV during exercise results in coefficients of variation of 3.3 and 4.1%, respectively (Chiappa et al. 2008a). Systolic arterial pressure was measured using the standard auscultatory technique and cardiac power output was estimated from the equation: cardiac power output = (Q T × SAP) × K, where SAP is the systolic arterial pressure, Q T is the cardiac output in and K the conversion factor 2.22 × 10−3 (Cooke et al. 1998).
Skeletal muscle oxygenation
Skeletal muscle oxygenation profiles of the left M. vastus lateralis were evaluated with a commercially available near-infrared spectroscopy (NIRS) system (Hamamatsu NIRO 200TM, Hamamatsu Photonics, Hamamatsu, Japan) as previously described (Borghi-Silva et al. 2008). A set of optodes was placed on the belly of the M. vastus lateralis midway between the lateral epicondyle and greater trochanter of the femur. The optodes were housed in an optically dense plastic holder, thus ensuring that the position of the optodes, relative to each other, was fixed and invariant. The optode assembly was secured on the skin surface with tape, and then covered with an optically dense, black vinyl sheet, thus minimizing the intrusion of extraneous light and loss of NIR light. The variables assessed by NIRS are the concentration of oxygenated, deoxygenated, and total hemoglobin. Among the NIRS variables, several laboratories have adopted the deoxy-Hb/Mb signal ([HHb]) as the preferred indicator of changes in muscle microvascular oxygenation during exercise (Borghi-Silva et al. 2008). The [HHb] response to exercise is then considered a proxy of O2 extraction in the microcirculation, reflecting the balance between O2 delivery and utilization. The values were recorded as a delta (Δ) from baseline in units of μM/cm and were expressed as % of the maximal value determined on a post-exercise maximal voluntary contraction.
Data analysis
The SPSS version 15.0 statistical software was used for data analysis (SPSS, Chicago, IL, USA). Results were summarized as mean ± SD. Between-intervention differences over time were compared by two-way analysis of variance with repeated measures. Where appropriate, multiple comparisons were performed with the Bonferroni post-hoc procedure. The level of statistical significance was set at P < 0.05 for all tests.
Results
Blood lactate and perceptual responses
Figure 1a shows the initial Wingate test resulted in significant increases in [Lac −b ]. Post-exercise peak [Lac −b ] was 12.5 ± 2.3 mmol l−1 and was significantly reduced to 9.8 ± 1.5 mmol l−1 with IRL. After the fourth minute of recovery, IRL resulted in significantly lower [Lac −b ] as reflected by the repeated measures ANOVA as well as by the analysis of the area under the curve (107 ± 15 with IRL vs. 146 ± 19 without resistance; P < 0.01). Therefore, pre-bout 2 [Lac −b ] was, on average, 27% less with IRL compared to control. Figure 1b shows the leg perception scores were reduced with IRL from the eighth minute to pre-bout 2 evaluation (15th min). Similarly, Fig. 1c shows dyspnea scores were lower with active intervention than control breathing in 8–10 min.
Mean ± SD arterialized blood lactate (panel A), leg perception scores (panel B), and breathlessness perception scores (panel C) during and after two sequential Wingate tests with (closed circles) and without (open circles) post-exercise inspiratory resistance loading. *P < 0.05: two-way analysis of variance with repeated measures, with multiple comparisons performed with the Bonferroni post-hoc procedure
Hemodynamics and peripheral muscle oxygenation
The Wingate test led to significant increases in HR, SV and Q T (Fig. 2a–c). Recovery IRL resulted in higher HR and QT, especially in the late recovery (Fig. 2a, c). These changes were accompanied by concomitant increases in systolic arterial pressure and cardiac power output with IRL (Fig. 2d, e, respectively). The Wingate test resulted in marked increases in peripheral muscle deoxygenation (Fig. 2f). Importantly, however, the rate of muscle reoxygenation during recovery was impaired with IRL: as shown in Fig. 2f, Δ [HHb] values were significantly higher with active intervention compared to control from the sixth minute to pre-bout 2 evaluation (P < 0.05).
Mean ± SD heart rate (HR; panel A), stroke volume (SV; panel B), cardiac output (QT; panel C), systolic arterial pressure (SAP; panel D), cardiac power output (CPO; panel E), and changes in deoxy-hemoglobin concentration (Delta[HHb], panel F) during and after two sequential Wingate tests with (closed circles) and without (open circles) post-exercise inspiratory resistance loading. *P < 0.05: two-way analysis of variance with repeated measures, with multiple comparisons performed with the Bonferroni post-hoc procedure
Subsequent exercise performance
Post-Wingate IRL resulted in improved performance on a subsequent exercise bout. Therefore, peak power on bout 2 was, on average, 25% higher after IRL compared to free-breathing. Moreover, the 5-s averaged power values during bout 2 (Fig. 3) and the AUCPW were both higher after IRL than control breathing (AUC = 16,830 ± 4,300 vs. 13,780 ± 4,899 a.u., respectively; P < 0.05). Post-Wingate IRL resulted in a worse fatigue index during the second bout (47.5 ± 10.0% in bout 1; 50.0 ± 11.4% in bout 2) while the second with free-breathing was associated with a smaller fatigue index (48.6 ± 9.9% in bout 1 vs. 43.0 ± 13.3 in bout 2; ANOVA P < 0.05, for interaction).
Mean ± SD power values during an initial Wingate test (bout 1) and a subsequent test (bout 2) performed after a 15-min recovery period in which the subjects breathed freely (open circles) or through an inspiratory resistance (closed circles). Two-way analysis of variance with repeated measures, (*P < 0.05 = bout 2 versus bout 1 with inspiratory resistance; †, inspiratory resistance versus control in bout 2 with multiple comparisons performed with the Bonferroni post-hoc procedure)
Discussion
The present study investigated the physiological and perceptual effects of IRL combined with passive leg recovery from all-out maximal-intensity exercise (Wingate test) in young healthy males. We extended our previous findings (Chiappa et al. 2008b) by showing that, besides reducing arterialized [Lac −b ] since early recovery, IRL had positive effects on post-exercise perception of leg effort and breathlessness despite evidences of impaired leg muscle reoxygenation. A discernible effect of IRL on indices of cardiac work was found just on late recovery, implying that myocardial Lac− uptake was not the main mechanism for Lac− clearance. In addition, IRL presented with a dual effect on subsequent performance to supra-maximal exercise, i.e., improvement in peak and mean power but increased fatigue index. This seems to constitute the first experimental evidence that post-exercise IRL can be of practical value in improving peak power on subsequent all-out maximal-intensity exercise and sets the stage for future large-scale, interventional studies in athletic populations exposed to repeated bouts of high-intensity exercise.
Short-term, high-intensity exercise is associated with a marked increase in La– production and slowed La– clearance, resulting on increased intramuscular [La–] and net La– output from muscles into the blood (Gladden 2004). The high oxidative potential of the respiratory muscles, especially the diaphragm, in association with their abundant perfusion (Fregosi and Dempsey 1986; Guenette et al. 2008), make these muscles particularly suited to promote net Lac− uptake following all-out maximal-intensity exercise. This view is strengthened by the findings of Fregosi and Dempsey (1986), who have shown that [Lac−] was elevated in the respiratory muscles during exercise in the absence of local glycogen utilization, suggesting increased [Lac−] uptake, rather than [Lac−] production. Moreover, Spengler et al. (1999) have previously shown that inspiratory muscle training results in reduced [Lac −b ] responses to exercise. In the present study, we confirm our previous observation that IRL during recovery from maximal incremental exercise results in lower [Lac −b ] (Chiappa et al. 2008b). As depicted in Fig. 1a, this effect was not trivial as IRL decreased [Lac −b ] by 27% compared to control breathing. In our previous study, the reduction in [Lac −b ] during recovery was not associated with changes in blood acid–base status, but resulted in increased metabolic demand and alveolar ventilation (Chiappa et al. 2008b). However, in that particular study we did not assess whether part of this effect could be ascribed to increased cardiac work. In the present study, we found that the reduction in [Lac −b ] with IRL occurred since early recovery (from 4 min) when between-treatment differences in cardiac output and cardiac power output were insignificant only during late recovery (Figs. 2c, e). Therefore, it is conceivable that the respiratory muscles were the main source of [Lac −b ] uptake during recovery.
There is a lively controversy on the beneficial effects of active recovery from exhausting exercise on the rate of Lac− clearance and performance on a subsequent test compared to passive recovery. Previous authors, for instance, found that activation of the exercised muscles in the recovery period can reduce [Lac −b ] and improve performance compared to passive recovery (Bishop et al. 2007; Bogdanis et al. 1996). In contrast, others have argued that active recovery may present with some deleterious consequences on the rate of muscle reoxygenation and phosphocreatine recovery kinetics (Dupont et al. 2004, 2007). An alternative approach to accelerate [Lac −b ] clearance, however, is the combination of passive recovery of the exercised muscles (usually those of the legs) with activation of other muscle groups that have not been directly involved in power output generation. In the present investigation, we found a significant increase in peak and mean power during the second exercise bout after IRL had reduced [Lac −b ] compared to free-breathing (Fig. 3). Our data suggest, but do not demonstrate, a mechanistic role for lower recovery [Lac −b ] in increasing tolerance to ensuing supra-maximal exercise, at least in the instantaneous rate of force production after exercise commencing. They also may justify previous positive effects of inspiratory muscle training on recovery time during high-intensity repetitive sprint tests, [Lac −b ] and perceptual responses to submaximal endurance exercise, and attenuation of peripheral muscle fatigue (McConnell and Lomax 2006; Romer et al. 2002). The negative effects of lactic acid accumulation on exercise performance are associated with proton accumulation (Allen et al. 2008). Since in our previous investigation we found no change in blood pH with IRL during recovery (Chiappa et al. 2008b), other mechanisms for the improvement in subsequent performance should be considered. More specifically, it is tempting to speculate that lower circulating [Lac −b ] levels during IRL could be related to a decreased sense of post-exercise effort and improved performance at the start of subsequent all-out exercise. However, more studies are needed to better evaluate the mechanisms by which IRL during recovery improves subsequent performance.
As previously shown (Nioka et al. 1998), the Wingate test resulted in marked increases in peripheral muscle deoxygenation (Fig. 2f). A particularly interesting finding of the present study was the significant reduction in the rate of post-exercise reoxygenation of the resting M. vastus lateralis with IRL compared to free-breathing, i.e., higher ΔHHb % from minute 6 onwards (Fig. 2f). As mentioned, [HHb] from a single site in the quadriceps has been widely used as a proxy of tissue ‘fractional’ O2 extraction (Ferreira et al. 2005). In this context, higher post-exercise (estimated) O2 extraction during IRL compared to free-breathing is likely to reflect a significant decrease in convective O2 delivery. The main hypothesis to explain these findings is that post-exercise IRL triggered the respiratory muscle metaboreflex. There are several lines of evidence suggesting that increased fatiguing contractions and accumulation of metabolites in the inspiratory and expiratory muscles may activate unmyelinated type IV phrenic afferents and some myelinated type III afferent fibers are also sensitive to local changes, increasing sympathetic vasoconstrictor activity with the purpose to redirect blood flow from peripheral to ventilatory muscles (Chiappa et al. 2008c; Harms et al. 1997; Sheel et al. 2001). In agreement with this concept, we found higher HR and mean arterial pressure with IRL, especially in the late recovery (Fig. 2a, d). As mentioned, this was associated with decreased O2 delivery as estimated by NIRS (Fig. 2f), even when QT was higher with IRL (Fig. 2c). Therefore, the IRL-triggered metaboreflex may have deviated blood flow from resting legs to respiratory muscles, minimizing the need of any further increase in Q T during recovery. Despite worse reoxygenation, leg effort perception scores were lower with IRL, most likely associated with the lower [Lac –b ].
In this context, it is interesting to note that potential activation of the metaboreflex and reduction in post-exercise O2 availability with IRL was associated with enhanced peak and mean power output on the subsequent Wingate test, but deterioration in the fatigue index. This is further complicated by the licit contention that relative peripheral muscle ischemia with IRL may have trapped Lac− within the exercised muscles and contributed to lower [Lac −b ] values. It is therefore conceivable that the beneficial effects of IRL on post-exercise perception scores (Fig. 1b, c) may have enhanced peak and mean power output on a subsequent volitional test. This is in agreement with the findings of Tong and Fu (2006), who have previously shown that specific inspiratory muscle warm-up reduces breathless sensation and enhances the performance of subsequent supra-maximal intermittent exercise. On the other hand, reduction in convective O2 delivery to the appendicular muscles during IRL may have contributed to accelerate the rate of decrement in muscle power output in bout 2.
In conclusion, IRL during recovery from all-out maximal-intensity exercise decreases [Lac −b ] and perception of leg effort with beneficial effects on peak and mean power on subsequent supra-maximal exercise in healthy males. Our results provide rationale for using post-exercise IRL as an ergogenic aid and future studies should be conducted to better evaluate the mechanisms responsible for this effect.
References
Allen DG, Lamb GD, Westerblad H (2008) Skeletal muscle fatigue: cellular mechanisms. Physiol Rev 88:287–332. doi:10.1152/physrev.00015.2007
Bishop D, Ruch N, Paun V (2007) Effects of active versus passive recovery on thermoregulatory strain and performance in intermittent-sprint exercise. Med Sci Sports Exerc 39:872–879. doi:10.1249/mss.0b013e318031b026
Bogdanis GC, Nevill ME, Lakomy HK, Graham CM, Louis G (1996) Effects of active recovery on power output during repeated maximal sprint cycling. Eur J Appl Physiol Occup Physiol 74:461–469. doi:10.1007/BF02337727
Borghi-Silva A, Carrascosa C, Oliveira CC, Barroco AC, Berton DC, Vilaça D, Lira-Filho EB, Ribeiro D, Nery LE, Neder JA (2008) Effects of respiratory muscle unloading on leg muscle oxygenation and blood volume during high-intensity exercise in chronic heart failure. Am J Physiol Heart Circ Physiol 294:H2465–H2472. doi:10.1152/ajpheart.91520.2007
Charloux A, Lonsdorfer-Wolf E, Richard R, Lampert E, Oswald-Mammosser M, Mettauer B, Geny B, Lonsdorfer J (2000) A new impedance cardiograph device for the non-invasive evaluation of cardiac output at rest and during exercise: comparison with the “direct” Fick method. Eur J Appl Physiol 82:313–3120. doi:10.1007/s004210000226
Chiappa GR, Borghi-Silva A, Ferreira LF, Carrascosa C, Oliveira CC, Maia J, Gimenes AC, Queiroga F Jr, Berton D, Ferreira EM, Nery LE, Neder JA (2008a) Kinetics of muscle deoxygenation are accelerated at the onset of heavy-intensity exercise in patients with COPD: relationship to central cardiovascular dynamics. J Appl Physiol 104:1342–1350. doi:10.1152/japplphysiol.01364.2007
Chiappa GR, Roseguini BT, Alves CN, Ferlin EL, Neder JA, Ribeiro JP (2008b) Blood lactate during recovery from intense exercise: impact of inspiratory loading. Med Sci Sports Exerc 40:111–116
Chiappa GR, Roseguini BT, Vieira PJC, Alves CN, Tavares A, Winkelmann ER, Ferlin EL, Stein R, Ribeiro JP (2008c) Inspiratory muscle training improves blood flow to resting and exercising limbs in patients with chronic heart failure. J Am Coll Cardiol 51:1663–1671. doi:10.1016/j.jacc.2007.12.045
Cooke GA, Marshall P, al-Timman JK, Wright DJ, Riley R, Hainsworth R, Tan LB (1998) Physiological cardiac reserve: development of a non-invasive method and first estimates in man. Heart 78:289–294
Dotan R (2006) The Wingate anaerobic test’s past and future and the compatibility of mechanically versus electro-magnetically braked cycle-ergometers. Eur J Appl Physiol 98:113–116. doi:10.1007/s00421-006-0251-4
Dupont G, Moalla W, Guinhouya C, Ahmaidi S, Berthoin S (2004) Passive versus active recovery during high-intensity intermittent exercises. Med Sci Sports Exerc 36:302–308. doi:10.1249/01.MSS.0000113477.11431.59
Dupont G, Moalla W, Matran R, Berthoin S (2007) Effect of short recovery intensities on the performance during two Wingate tests. Med Sci Sports Exerc 39:1170–1176. doi:10.1249/mss.0b013e31804c9976
Ferreira LF, Townsend DK, Lutjemeier BJ, Barstow TJ (2005) Muscle capillary blood flow kinetics estimated from pulmonary O2 uptake and near-infrared spectroscopy. J Appl Physiol 98:1820–1828. doi:10.1152/japplphysiol.00907.2004
Fregosi RF, Dempsey JA (1986) Effects of exercise in normoxia and acute hypoxia on respiratory muscle metabolites. J Appl Physiol 60:1274–1283. doi:10.1063/1.337350
Gladden LB (2004) Lactate metabolism: a new paradigm for the third millennium. J Physiol 558:5–30. doi:10.1113/jphysiol.2003.058701
Guenette JA, Vogiatzis I, Zakynthinos S, Athanasopoulos D, Koskolou M, Golemati S, Vasilopoulou M, Wagner HE, Roussos C, Wagner PD, Boushel R (2008) Human respiratory muscle blood flow measured by near-infrared spectroscopy and indocyanine green. J Appl Physiol 104:1202–1210. doi:10.1152/japplphysiol.01160.2007
Hamilton AL, Killian KJ, Summers E, Jones NL (1995) Muscle strength, symptom intensity, and exercise capacity in patients with cardiorespiratory disorders. Am J Respir Crit Care Med 152:2021–2031
Harms CA, Babcock MA, McClaran SR, Pegelow DF, Nickele GA, Nelson WB, Dempsey JA (1997) Respiratory muscle work compromises leg blood flow during maximal exercise. J Appl Physiol 18:480–486
Hill AV, Long CNH, Lupton H (1924) Muscular exercise, lactic acid, and the supply and utilization of oxygen. Part VI. The oxygen debt at the end of exercise. Proc R Soc Lond B Biol Sci 97:127–137
McConnell AK, Lomax M (2006) The influence of inspiratory muscle work history and specific inspiratory muscle training upon human limb muscle fatigue. J Physiol 577:445–457. doi:10.1113/jphysiol.2006.117614
Nioka S, Moser D, Lech G et al (1998) Muscle deoxygenation in aerobic and maximal-intensity exercise. Adv Exp Med Biol 454:63–70
Romer LM, McConnell AK, Jones DA (2002) Effects of inspiratory muscle training upon recovery time during high intensity, repetitive sprint activity. Int J Sports Med 23:353–360. doi:10.1055/s-2002-33143
Sheel AW, Derchak PA, Morgan BJ, Pegelow DF, Jacques AJ, Dempsey JA (2001) Fatiguing inspiratory muscle work causes reflex reduction in resting leg blood flow in humans. J Physiol 537:277–289. doi:10.1111/j.1469-7793.2001.0277k.x
Spengler CM, Roos M, Laube SM, Boutellier U (1999) Decreased exercise blood lactate concentrations after respiratory endurance training in humans. Eur J Appl Physiol 79:299–305. doi:10.1007/s004210050511
Tong TK, Fu FH (2006) Effect of specific inspiratory muscle warm-up on intense intermittent run to exhaustion. Eur J Appl Physiol 97:673–680. doi:10.1007/s00421-006-0233-6
Acknowledgments
The authors would like to thank Prof. Luiz E. Nery (Division of Respiratory Diseases. Department of Medicine, Federal University of Sao Paulo, UNIFESP) for his valuable input and methodological insights. They also thank all colleagues from the Pulmonary Function and Clinical Exercise Physiology Unit (SEFICE, UNIFESP) for their friendly collaboration. They are specifically grateful for the support of Ana Cristina Gimenes, MSc, PT, Ana Cristina Barroso, PT, Daniela Bravo, PT, and Ms. Dircilene P. Moreira. More importantly, however, they are indebted to the individuals for their effort and enthusiastic cooperation throughout the study. GRC is a Post-Doctoral Researcher at the Federal University of São Paulo, supported by a Fellowship Grant from FAPESP (Fundação de Amparo à Pesquisa do Estado de Sao Paulo, São Paulo, Brazil) No 07/50391-5. JAN is an Established Investigator (level II) of the Conselho Nacional de Desenvolvimento Científico e Tecnológico (CNPq), Brazil.
Conflict of interest statement
The authors report no potential conflict of interest related to the content of this article.
Author information
Authors and Affiliations
Corresponding author
Rights and permissions
About this article
Cite this article
Chiappa, G.R., Ribeiro, J.P., Alves, C.N. et al. Inspiratory resistive loading after all-out exercise improves subsequent performance. Eur J Appl Physiol 106, 297–303 (2009). https://doi.org/10.1007/s00421-009-1022-9
Accepted:
Published:
Issue Date:
DOI: https://doi.org/10.1007/s00421-009-1022-9