Abstract
Control of intestinal motility requires an intact enteric neurotransmission. Synaptosomal-associated protein 25 (SNAP-25) is an essential component of the synaptic vesicle fusion machinery. The aim of the study was to investigate the localization and expression of SNAP-25 in the human intestine and cultured enteric neurons and to assess its regulation by the neurotrophic factor glial cell line-derived neurotrophic factor (GDNF). SNAP-25 expression and distribution were analyzed in GDNF-stimulated enteric nerve cell cultures, and synaptic vesicles were evaluated by scanning and transmission electron microscopy. Human colonic specimens were processed for site-specific SNAP-25 gene expression analysis and SNAP-25 immunohistochemistry including dual-labeling with the pan-neuronal marker PGP 9.5. Additionally, gene expression levels and distributional patterns of SNAP-25 were analyzed in colonic specimens of patients with diverticular disease (DD). GDNF-treated enteric nerve cell cultures showed abundant expression of SNAP-25 and exhibited granular staining corresponding to synaptic vesicles. SNAP-25 gene expression was detected in all colonic layers and isolated myenteric ganglia. SNAP-25 co-localized with PGP 9.5 in submucosal and myenteric ganglia and intramuscular nerve fibers. In patients with DD, both SNAP-25 mRNA expression and immunoreactive profiles were decreased compared to controls. GDNF-induced growth and differentiation of cultured enteric neurons is paralleled by increased expression of SNAP-25 and formation of synaptic vesicles reflecting enhanced synaptogenesis. The expression of SNAP-25 within the human enteric nervous system and its downregulation in DD suggest an essential role in enteric neurotransmission and render SNAP-25 as a marker for impaired synaptic plasticity in enteric neuropathies underlying intestinal motility disorders.
Similar content being viewed by others
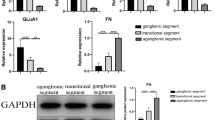
Avoid common mistakes on your manuscript.
Introduction
The human enteric nervous system (ENS) represents the largest neuronal network outside the central nervous system (CNS) with more than 150 million neurons extending throughout the entire gastrointestinal (GI) tract (Costa and Brookes 1994). The ENS regulates intestinal motility and various other functions in the GI tract, such as blood flow, mucosal transport, secretion, immunological and endocrine functions as well as water and ion transport (Costa et al. 2000; Snoek et al. 2010). Components mediating GI motility include the ENS as initiator that transmits the signals to the effector organ, the enteric smooth musculature, via neurotransmitters and their receptors (Furness 2006, 2012).
In neurons, these neurotransmitters are stored in synaptic vesicles that are released upon excitation by exocytosis. The neurotransmitter release machinery in eukaryotes requires soluble N-ethylmaleimide-sensitive factor attachment protein receptor (SNARE) proteins for synaptic vesicle fusion. SNARE proteins can be divided into vesicle or v-SNAREs (e.g., synaptobrevin), which are incorporated into the membranes of transport vesicles during budding, and target or t-SNAREs, which are located in the membranes of target compartments. t-SNAREs include the membrane-associated proteins syntaxin and 25 kDa synaptosomal-associated protein (SNAP-25) that form complexes between the synaptic vesicle and cell membranes (Jahn and Scheller 2006; Rizo and Südhof 2002). Moreover, SNAP-25 also modulates different voltage-gated calcium channels (Catterall 1999; Zamponi 2003). While the role of SNAP-25 in the CNS has been extensively studied, little data are available concerning SNAP-25 expression and localization in the human and rat ENS. Previous studies have used SNAP-25 as pan-axonal marker of the ENS in murine cecum and colon (Lourenssen et al. 2010; Poli et al. 2001) or to characterize membrane specialization between nerve terminals and intestinal cells of Cajal in the murine stomach (Beckett et al. 2005). However, a systematic investigation of SNAP-25 in the adult ENS is still missing.
The physiological importance of the synaptic vesicle apparatus and its delicate interplay with the presynaptic membrane becomes evident under pathophysiological conditions as described for the central nervous system (CNS) (Benarroch 2013; Südhof and Rizo 2011). Recent research on neurodegenerative diseases of the CNS could demonstrate the impact of synaptic dysfunction as an initial event of subsequent neurodegeneration (Burgoyne and Morgan 2011). Of note, the reduction of SNAP-25 levels was observed before the onset of neurodegeneration (Sharma et al. 2012), making SNAP-25 a putative candidate to be used for early diagnosis and prevention of neurodegenerative diseases.
Neurotrophic factors play a critical role in maturation, maintenance and survival of the ENS not only during development but also for the postnatal period (Schäfer and Mestres 1999; von Boyen et al. 2002). Glial cell line-derived neurotropic factor (GDNF), originally isolated from cell culture supernatants of a glioblastoma cell line (Lin et al. 1993), acts as a potent neurotrophic factor for the ENS. Its signal transduction is mediated by the tyrosine kinase receptor “rearranged during transfection” (RET) and the GDNF family receptor alpha 1 (GFRα1), a glycosyl-phosphatidylinositol-anchored protein (Airaksinen and Saarma 2002). Studies on cultures of postnatal myenteric neurons revealed significant effects of the GDNF system on neuronal survival and neurite outgrowth at later stages of ENS development (Rodrigues et al. 2011; Schäfer and Mestres 1999). Moreover, a study found evidences for GDNF to promote rodent hippocampal synaptogenesis (Ledda et al. 2007). Recently, a deficiency of the enteric GDNF system could be shown in diverticular disease (Böttner et al. 2013a)—a condition which is associated with intestinal motility disturbances and a decline of enteric nerve cells as well as alterations of several enteric neurotransmitter systems (Wedel and Böttner 2014).
Both neuronal survival/maintenance and synaptic plasticity/neurotransmission can be impaired in enteric neuropathies underlying intestinal motility disorders (Knowles et al. 2010). Although diagnostic criteria for histopathological diagnosis of gastrointestinal neuromuscular pathology have been released only recently (Knowles et al. 2009), the characterization of essential components of the synaptic vesicle apparatus may give further insights into the pathophysiological mechanisms of disturbed intestinal motor functions and provide novel diagnostic tools. Thus, the aim of the study was to investigate the localization and expression of SNAP-25 in cultured enteric neurons, to assess its regulation by the neurotrophic factor GDNF and to monitor the formation of synaptic vesicles. Moreover, site-specific gene expression levels and distributional pattern of SNAP-25 were studied in the human GI tract. Finally, since diverticular disease (DD) is associated with a gastrointestinal neuromuscular pathology including a deficiency of the GDNF system, SNAP-25 mRNA expression and immunoreactive signals were analyzed in patients with DD and compared to controls.
Materials and methods
Enteric nerve cell cultures
Generation of enteric nerve cell cultures
Preparation of myenteric nerve cells was performed according to a method described previously (Schäfer et al. 1997). Briefly, after removing the small intestine from newborn Wistar rats (postnatal day 2–3), the tunica muscularis was stripped from the mucosa, followed by the incubation for 2 h at 37 °C in Ca2+- and Mg2+-free Hanks’ balanced salt solution (HBSS, Gibco Life Technologies/Invitrogen, Karlsruhe, Germany) with antibiotics containing 1 mg/ml collagenase (SIGMA, Munich, Germany). Afterward, fragments of myenteric plexus were collected under stereomicroscopic control and incubated for 15 min at 37 °C in trypsin/EDTA (0.125 mg/ml Gibco, Life Technologies, Germany) to dissociate the plexus. The procedure was stopped by replacing trypsin/EDTA with fetal calf serum (FCS, Gibco, Life Technologies/Invitrogen, Karlsruhe, Germany). The cells were triturated, counted and seeded in a density of 100,000 cells/ml on poly-D-Lysin-(SIGMA)-/Laminin (SIGMA, Munich, Germany)-coated coverslips for immunocytochemistry studies or 12-well plates for gene expression studies. Cells were incubated in defined medium consisting of Neurobasal A (Gibco, Life Technologies/Invitrogen, Karlsruhe, Germany) and B27 supplement (Gibco, Life Technologies/Invitrogen, Karlsruhe, Germany). Additionally, GDNF (Peprotech, Hamburg, Germany) was added to a final concentration of 2, 10 or 50 ng/ml. For gene expression analysis, cells were cultured for 1 week; for immunocytochemical and electronmicroscopical analysis, culture time was 3 weeks. Medium was changed twice a week.
Immunocytochemistry in enteric nerve cell cultures
Cells were fixed for 30 min with 4 % paraformaldehyde, permeabilized for 10 min with methanol and treated for 10 min with 3 % H2O2. After blocking of unspecific background with normal goat serum (1:10, DakoCytomation, Glostrup, Denmark) for 30 min, incubation with a mouse anti-PGP 9.5 antibody (1:1,000, Acris, Herford, Germany) for 1 h was performed, followed by incubation with a biotinylated secondary antibody (goat anti-mouse IgG, 1:400) for 45 min and treatment with an avidin–biotin-complex (Vectastain ABC Standard, Vector Laboratories, Burlingame, CA) conjugated with horseradish peroxidase for 45 min. Antibody binding was visualized with 3,3′-diaminobenzidine (DAB, DakoCytomation, Glostrup, Denmark). Analysis was carried out with a light optical microscope (Nikon 6000, Nikon, Tokyo) coupled to a digital camera (Digital Sight, Nikon, Tokyo). Data acquisition was performed with NIS-Elements BR 3.2 software (Nikon, Tokyo).
For dual-label immunocytochemistry for SNAP-25 and PGP 9.5, cells were fixed for 30 min with 4 % paraformaldehyde, permeabilized for 10 min with methanol and treated for 10 min with 3 % H2O2. Afterward, incubation with a rabbit anti-SNAP-25 antibody (1:100, Acris, Herford, Germany) and a mouse anti-PGP 9.5 antibody (1:1,000, Acris, Herford, Germany) for 1 h was performed, followed by incubation with the secondary antibodies, goat anti-rabbit-AlexaFluor488 (1:250, Invitrogen, Life technologies, Carlsbad, CA, USA) and goat anti-mouse-AlexaFluor546 (1:250, Invitrogen, Life technologies, Carlsbad, CA, USA). Finally, cells were counterstained with DAPI (Roche, Mannheim, Germany) to visualize cell nuclei. Analysis was carried out with a fluorescent microscope (Axiovert 200 M, Zeiss, Göttingen, Germany) coupled to a digital camera (Axiocam, Zeiss, Göttingen, Germany). Data acquisition was performed with Axiovision software (Zeiss, Göttingen, Germany).
Gene expression studies in enteric nerve cell cultures
Extraction of RNA from enteric nerve cell cultures was performed using the Nucleospin XS kit (Macherey and Nagel, Düren, Germany) according to the manufacturer’s guidelines. RNA was eluted in a volume of 15 μl H2O. Genomic DNA was digested for 15 min at room temperature using 1.5 U of DNase I (Sigma-Aldrich, Munich, Germany). Reverse transcription was carried out in a total volume of 30 μl containing 375 ng random hexamer primer (GE Healthcare, Freiburg, Germany), 0.5 mM dNTPs (Promega, Mannheim, Germany), 0.01 M DTT, 1× reaction buffer and 150 U Superscript II Reverse Transcriptase (Invitrogen, Karlsruhe, Germany). The annealing, elongation and denaturation steps were carried out at 25 °C for 10 min, at 42 °C for 50 min and at 70 °C for 15 min, respectively. Reverse transcription and qPCR were performed as described before (Böttner et al. 2010). Forward and reverse primers as well as probes are listed below.
Rat snap25: forward primer: 5-ggcttcatccgcagggtaac-3′, reverse primer: 5′-agggccatatgacggaggtt-3′, probe: 5-acgatgcccgggaaaatgaaatgga-3′.
Rat HPRT (housekeeping gene): forward primer: 5′-cgccagcttcctcctcaga-3′, reverse primer: 5′-ggtcataacctggttcatcact-3′, probe: 5′-ttttcccgcgagccgaccgg-3′.
Scanning electron microscopy of enteric nerve cells cultures
After 3 weeks, cultured enteric neurons treated with GDNF (50 ng/ml) were fixed by 3 % glutaraldehyde in PBS for 30 min, followed by postfixation for 20 min in 2 % osmiumoxide and dehydration in increasing series of ethanol. After that, probes were critical-point dried (CPD 030, Balzers) and sputtered with gold (Ion Tech LTD). Analysis was performed by using a scanning electron microscope (Phillips XL 20, Phillips).
Transmission electron microscopy of enteric nerve cell cultures
After 3 weeks, cultured enteric neurons treated with GDNF (50 ng/ml) were fixed in 3 % glutaraldehyde in PBS for 30 min. After postfixation in 2 % osmiumoxide for 2 × 15 min and dehydration in increasing series of ethanol, cultured cells growing on coverslips were embedded in araldite overnight. Coverslips were carefully removed from the araldite block which was directly trimmed for ultra-thin sectioning. Ultra-thin sections (40–50 nm) were cut (Ultracut, Reichert-Jung, Germany) parallel to the surface of the cultured cellular network using a diamond knife and were contrasted with uranyl acetate for 15 min as well as lead citrate for 7 min. Analysis was carried out with a transmission electron microscope (EM 900, Zeiss) coupled to a digital imaging system (Slow Scan CCD-Camera, type 7888-IV, TRS).
Human GI tissue
Site-specific expression pattern and immunohistochemistry
Colonic full-thickness sections were obtained from patients who underwent sigmoid resection or left hemicolectomy for non-obstructive colorectal carcinoma (n = 8, 8 males, age range 48–86 years). Patients reported normal bowel habits and showed no evidence of anorectal outlet obstruction. After surgical removal, specimens were harvested at safe distance (>5 cm) from the tumor site.
Comparative study
Colonic full-thickness sections were obtained from patients who underwent sigmoid resection or left hemicolectomy for non-obstructive colorectal carcinoma (n = 10, 4 females, 6 males, age range 48–90 years). Patients reported normal bowel habits and showed no evidence of anorectal outlet obstruction. After surgical removal, specimens were harvested at safe distance (>5 cm) from the tumor site.
Full-thickness sections of sigmoid colon from patients with diverticular disease (DD) were obtained (n = 9, 7 females, 2 males, age range 49–74 years) after sigmoid resection or left hemicolectomy for DD. Patients were operated after two or more attacks of diverticulitis by elective surgery. Specimens were harvested from sites adjacent to colonic diverticula. Diverticula-containing areas displaying an altered anatomy of the colonic wall due to transmural mucosal/submucosal outpouchings or signs of inflammation and fibrotic scaring were excluded from tissue sampling.
All specimens were immediately transferred from the operating room to the laboratory for tissue processing. The study of human tissue received approval from the Ethics Committee of the Faculty of Medicine, Christian-Albrecht’s University in Kiel, Germany (B 299/07), and has been carried out in accordance with “The Code of Ethics of the World Medical Association.”
Immunocytochemistry in human GI tissue
Full-thickness tissue blocks were trimmed to a size of 30 mm × 10 mm, pinned out flat and tension-free on a cork plate and fixated with 4 % paraformaldehyde in PBS for 24 h. After dehydration, the tissue blocks were transferred into paraffin wax and cut orthogonally in 6-µm sections. The cutting surface (30-mm border) was orientated perpendicular to the gut axis and parallel to the circular muscle layer. Visualization of SNAP-25 was achieved by immunohistochemistry using primary mouse anti-SNAP-25 antibody (1:10,000, Lifespan Biosci., Seattle, WA, USA). Immunohistochemistry was performed as described previously (Böttner et al. 2012). Briefly, pretreatment was performed by incubating the sections with citrate buffer (pH 6.0, microwaves 750 W) for 10 min. Afterward, samples were incubated overnight with primary antibodies diluted in antibody diluent (Zymed, Invitrogen, Life technologies, Carlsbad, CA, USA) followed by incubation with biotinylated secondary antibodies (goat anti-mouse IgG, 1:400, DakoCytomation, Glostrup, Denmark) for 45 min. Thereafter, samples were treated with an avidin–biotin-complex (Vectastain ABC Standard, Vector Laboratories, Burlingame, CA) conjugated with horseradish peroxidase for 45 min. Antibody binding was visualized with 3,3′-diaminobenzidine (DAB, DakoCytomation, Glostrup, Denmark). Additionally, counterstaining with hematoxylin was performed. Analysis was carried out with a light optical microscope (Nikon 6000, Nikon, Tokyo) coupled to a digital camera (Digital Sight, Nikon, Tokyo). Data acquisition was performed with NIS-Elements BR 3.2 software (Nikon, Tokyo).
To demonstrate neuronal localization of SNAP-25, co-staining with the pan-neuronal marker PGP 9.5 was performed. After pretreatment with citrate buffer (pH 6.0, 750 W microwaves for 2 × 5 min), sections were incubated overnight with mouse anti-SNAP-25-antibodies (1:10,000, Lifespan, Seattle, USA) diluted in antibody diluent (Invitrogen, Carlsbad, USA). Afterward, sections were incubated for 2 h at room temperature with a goat anti-mouse AlexaFluor488 antibody (1:250, Invitrogen, Karlsruhe, Germany). To visualize neuronal localization, co-incubation with rabbit PGP 9.5-antibody (1:2,000, UCL, Isle of Wight, GB) was performed followed by incubation with goat anti-rabbit AlexaFluor546 antibody (1:250, Invitrogen, Karlsruhe, Germany) for 2 h at room temperature. Finally, DAPI (Roche, Mannheim, Germany) was used to visualize cellular nuclei. Analysis was carried out with a fluorescence microscope (Axiovert 200 M, Zeiss, Jena, Germany) coupled to a digital camera (Axiocam, Zeiss, Jena, Germany). Data acquisition was performed using Axiovision software (Zeiss, Jena, Germany).
Gene expression studies in human GI tissue
Extraction of total RNA from the human colon (full-thickness sections, tunica muscularis, mucosa) and from human myenteric ganglia harvested by laser capture microdissection (LMD) was performed using the peqGOLD MicroSpin Total RNA Kit (PEQLAB, Erlangen, Germany) according to the manufacturer’s instructions. RNA was eluted in a volume of 15 μl H2O. Genomic DNA was digested for 15 min at room temperature using 1.5 U of DNase I (Sigma-Aldrich, Munich, Germany). Reverse transcription was carried out in a total volume of 30 μl containing 375 ng random hexamer primer (GE Healthcare, Freiburg, Germany), 0.5 mM dNTPs (Promega, Mannheim, Germany), 0.01 M DTT, 1× reaction buffer and 150 U Superscript II Reverse Transcriptase (Invitrogen, Karlsruhe, Germany). The annealing, elongation and denaturation steps were carried out at 25 °C for 10 min, at 42 °C for 50 min and at 70 °C for 15 min, respectively. Reverse transcription and qPCR were performed as described before (Bottner et al. 2010). Forward and reverse primers as well as probes are listed below:
Human SNAP-25: forward primer: 5′-ggacgaacgggagcagatg-3′, reverse primer: 5′-cgctcacctgctctaggtttc-3′, probe: 5′-tccgcagggtaacaaatgatgcccg-3′.
Human HPRT (housekeeping gene): forward primer: 5′-tgaacgtcttgctcgagatgtg-3, reverse primer: 5′-ccagcaggtcagcaaagaattt-3′, probe: 5′-tgggaggccatcacattgtagcc-3′.
Laser capture microdissection (LMD) of human myenteric ganglia
As described previously (Bottner et al. 2010), full-thickness specimens were immediately frozen in isopentane and stored at −70 °C until use. Cryosections (14 µm) were placed on membrane-coated slides (polyethylene naphthalate, 1 µm, Carl Zeiss MicroImaging GmbH, Göttingen, Germany), and regions of interest were visualized by ultra-rapid (ca. 30 s) staining with cresyl violet according to manufacturer’s instructions (PALM RNA Handling Protocols, Zeiss MicroImaging, Göttingen, Germany). Myenteric ganglia were identified by inverse light microscopy (Axiovert, Zeiss, Jena, Germany), excised and collected by LMD (P.A.L.M. Microlaser Technologies, Bernried, Germany) in the cap of 0.5-ml reaction tubes. From each sample, 2-mm2 ganglionic tissue was collected, immediately dissolved in 200 μl RNA lysis buffer (PEQLAB, Erlangen, Germany) and stored at −70 °C.
Statistical analysis
Gene expression studies were analyzed by Student’s t test (PrismTM, GraphPad, Sand Diego, CA, USA), and p values were corrected for multiple comparisons according to the false-discovery rate procedure, using R 2.13.1 (R-Core-Team 2012). Differences were considered significant if p < 0.05.
Results
Effects of GDNF on neuronal network formation of cultured enteric neurons
To assess the impact of GDNF treatment on neuronal network formation of cultured enteric neurons, we assessed GDNF-treated cultures (50 ng/ml) and controls by performing immunocytochemistry for the pan-neuronal marker PGP 9.5. After a culture time of 1 week, accumulation of neuronal somata and pronounced sprouting of nerve fibers were observed following GDNF treatment (data not shown, for further details see Böttner et al. 2013a). During prolonged culture time, nerve cell loss and atrophy of nerve fibers were obvious in untreated controls. After 3-week culture time, only sparse nerve cell aggregates and nerve fibers could be found in controls (Fig. 1a), whereas GDNF-treated cultures developed extensive neuronal networks characterized by large-sized nerve cell aggregates resembling enteric ganglia and interconnecting nerve fiber bundles (Fig. 1b).
Enteric nerve cell cultures form neuronal networks after GDNF treatment. Rat enteric nerve cells were cultured for 3 weeks. In contrast to untreated cultures (a), treatment with GDNF (50 ng/ml) induced the formation of neuronal networks composed of regularly distributed nerve cell aggregations (arrows) and interconnecting nerve fibers (arrowheads) (b). Immunocytochemistry for PGP 9.5. Scale bars 100 µm
Effects of GDNF on snap25 mRNA expression in cultured enteric neurons
To investigate the effect of the neurotropic factor GDNF on gene regulation of snap25, mRNA expression of snap25 was measured by quantitative RT-PCR in rat enteric nerve cell cultures treated with increasing concentrations of GDNF (Fig. 2). After GDNF treatment for 1 week, mRNA expression of snap25 was significantly upregulated in cultures treated with either 2 ng/ml GDNF or 50 ng/ml GDNF compared to untreated controls. The average mRNA expression of snap25 in GDNF-treated cultures was fivefold upregulated in cultures treated with 2 ng/ml GDNF and tenfold upregulated in cultures treated with 50 ng/ml GDNF compared to untreated controls. Due to the high variance, effects in cultures treated with 10 ng/ml GDNF turned out to be slightly outside the level of significance (p = 0.055).
mRNA expression of snap25 in enteric nerve cell cultures in response to GDNF treatment. GDNF increases mRNA levels of snap25 in enteric nerve cells cultured for 6 days. Expression levels of the target gene were normalized to expression of the housekeeping gene HPRT. Data are shown as mean ± SEM, n = 11–14, p < 0.05 versus control
Localization of SNAP-25 in cultured enteric neurons
To assess the localization pattern of SNAP-25 in cultured enteric neurons, dual-label immunohistochemistry for SNAP-25 (Fig. 3b, c) and PGP 9.5 (Fig. 3a, c) was performed after GDNF treatment (50 ng/ml) for 3 weeks. A clear neuronal localization of SNAP-25 (Fig. 3c) could be confirmed. While the pan-neuronal marker PGP 9.5 displayed an uniform labeling of neuronal elements, a distinct granular staining pattern of SNAP-25 was detectable in nerve cell aggregates (arrows in Fig. 3c) as well as in interconnecting nerve fibers (arrowheads in Fig. 3c). These granular SNAP-25 immunoreactive signals most likely represent neuronal varicosities as sites of neurotransmitter release.
Neuronal localization of SNAP-25 in enteric nerve cell cultures. Dual-label immunocytochemistry for the pan-neuronal marker PGP 9.5 (a) and SNAP-25 (b) illustrates neuronal localization of SNAP-25 (c) in enteric nerve cells cultured for 3 weeks and treated with GDNF (50 ng/ml). SNAP-25 immunoreactive signals display a distinct granular staining pattern of neuronal somata (arrows) and interconnecting nerve fibers (arrowheads). DAPI staining of nuclei. Scale bars 50 µm
Electron microscopy of enteric nerve cell cultures
Scanning electron microscopy was used to analyze the surface morphology of cultured neuronal networks. After GDNF treatment (50 ng/ml) for 3 weeks, a delicate neuronal network was discernible consisting of regularly distributed nerve cell aggregates and interconnecting nerve fiber strands (Fig. 4a). Higher magnification revealed frequent and distinct oval thickenings (ca. 1 µm diameter) along nerve fiber strands most likely resembling neuronal varicosities harboring accumulated synaptic vesicles (Fig. 4b).
Scanning electron microscopy of enteric nerve cell cultures. Rat enteric nerve cells cultured for 3 weeks with GDNF (50 ng/ml) form complex neuronal networks (a). At higher magnifications, multiple round thickenings along enteric nerve fiber strands are discernible most likely representing preterminal synaptic varicosities (arrows in b). Scale bars 200 µm (a), 2 µm (b)
Further evidence for the presence of neuronal varicosities along nerve fiber strands was given by transmission electron microscopy. At regular intervals, an axonal thickening or “swelling” could be observed containing grouped synaptic vesicles. Neuronal varicosities displayed a heterogeneous spectrum of large-sized, electron-dense synaptic vesicles and small-sized, electron-lucent synaptic vesicles (Fig. 5). The size of synaptic vesicles ranged between 30 and 100 nm.
Transmission electron microscopy of neuronal varicosities. Transmission electron microscopy of rat enteric nerve cells, cultured for 3 weeks with GDNF (50 ng/ml), reveals axonal thickenings or “swellings” containing a heterogeneous spectrum of large-sized, electron-dense synaptic vesicles (arrowhead) and small-sized, electron-lucent synaptic vesicles (arrow). The size of synaptic vesicles ranged between 30 and 100 nm. Scale bar 1 µm
Site-specific snap25 mRNA expression in the human colon
mRNA expression of snap25 was detectable in full-thickness sections of the human colon as well as in separate intestinal layers (tunica muscularis, mucosa) (Fig. 6a). Highest expression values were yielded within the tunica muscularis composed of the circular and longitudinal musculature and harboring the largest ganglionic nerve plexus. To further confirm site-specific snap25 mRNA expression within the myenteric plexus, myenteric ganglia were harvested by LMD. Gene expression analysis of myenteric ganglionic tissue yielded high expression profile for snap25 (Fig. 6b).
Site-specific mRNA expression of SNAP-25 in the human colon. mRNA expression of SNAP-25 in the human colon is detectable in full-thickness sections (FT-S), tunica muscularis (TM) and mucosa (MUC) (a) as well as in laser-microdissected myenteric ganglia (PM) (b) with highest expression values within the PM. Data are normalized to expression levels of the housekeeping gene HPRT and presented as mean ± SEM, a n = 5, b n = 8
Localization and distribution of SNAP-25 in the human colon
Immunohistochemistry using DAB as chromogene was applied to colonic full-thickness sections to assess the localization and staining pattern of SNAP-25. Robust and strong immunoreactive signals of SNAP-25 could be detected within the entire components of the ENS including submucosal and myenteric ganglia as well as nerve fibers running within the circular and longitudinal muscle layer (Fig. 7). SNAP-25 immunoreactivity in neuronal somata appeared to be less pronounced compared to the surrounding neuropil. At higher magnifications, a small-sized granular staining pattern could be observed in the ganglionic neuropil and along intramuscular nerve fibers (Fig. 7).
Localization of SNAP-25 in the human colon. Robust and strong immunoreactive signals of SNAP-25 (brown color) are detectable within the entire ENS including submucosal (a, b) and myenteric ganglia (c, d) as well as nerve fibers running within the circular (e) and longitudinal muscle layer (f). SNAP-25 immunoreactivity in neuronal somata (arrows in b and d) appears to be less pronounced compared to the surrounding neuropil. A small-sized granular distribution pattern is discernible within ganglia and nerve fibers at higher magnifications (b–f). Muc mucosa, SM submucosa, CM circular muscle layer, LM longitudinal muscle layer, SMP submucosal plexus, MP myenteric plexus, N neuronal somata, NF nerve fibers. Hematoxylin counterstain. Scale bars 100 µm (a–f)
To further prove neuronal localization of SNAP-25, dual-label immunocytochemistry with the pan-neuronal marker PGP 9.5 was performed. Co-localization of SNAP-25 and PGP 9.5 was detectable both within enteric ganglia and nerve fibers indicating a specific neuronal expression of SNAP-25 in the ENS (Fig. 8). Again, a distinct granular staining pattern of SNAP-25 was discernible in the ganglionic neuropil surrounding neuronal somata and along enteric nerve fibers (Fig. 8).
Co-localization of SNAP-25 and PGP 9.5 in the human colon. SNAP-25 immunoreactivity (green) is detectable in enteric nerve cells visualized by the pan-neuronal marker PGP 9.5 (red) of myenteric ganglia (arrows in a–c) as well as in enteric nerve fibers of the circular muscle layer (arrowhead in d–f). Immunoreactive signals of SNAP-25 are stronger in the neuropil (arrowhead in a, c) and enteric nerve fibers (arrowhead in d–f) compared to neuronal somata. SNAP-25 shows a distinct granular staining pattern in the neuropil, particularly around neuronal somata (a, c) and along enteric nerve fibers (d, f). Blue color DAPI staining of nuclei. Scale bars 50 µm (a–f)
Changes in SNAP-25 immunoreactivity and snap25 mRNA expression in diverticular disease (DD)
As DD has been reported to be associated with an enteric neuropathy (Wedel et al. 2010) and an impaired GDNF system (Böttner et al. 2013a), alterations of SNAP-25 expression and distribution were analyzed in patients with DD and compared to controls. As already shown in Fig. 7, robust and strong SNAP-25 immunoreactive signals were detected in enteric ganglia and nerve fibers obtained from controls (Fig. 9a), while patients with DD displayed considerably decreased and patchy immunoreactive signals within myenteric ganglia (Fig. 9b). The weakened immunoreactivity affected both neuronal somata and the ganglionic neuropil. Accordingly, mRNA expression of SNAP-25 was significantly decreased in the tunica muscularis of patients with DD with a nearly 36-fold mRNA reduction compared to controls (Fig. 9c).
SNAP-25 is decreased in patients with DD. Robust SNAP-25 immunoreactive signals (brown color) detected in myenteric ganglia of controls (a) are reduced in both neuronal somata and the neuropil of myenteric ganglia from patients with DD (b). Scale bars 120 µm. Confirmatory, the mRNA expression of SNAP-25 is decreased in the tunica muscularis of patients with DD compared to controls (c). Data are normalized to expression levels of the housekeeping gene HPRT and presented as mean ± SEM, n = 10 (controls) and n = 9 (DD). *p < 0.05 versus control
Discussion
Role of SNAP-25 in the CNS and ENS
Communication in the nervous system requires an intact synaptic transmission involving the release of neurotransmitters mediated by exocytosis of synaptic vesicles at the presynaptic active zone of nerve terminals (Südhof 1995, 2004). In the CNS, SNAP-25 contributes to the exocytosis of synaptic vesicles and numerous studies have implicated the involvement of SNAP-25 in the release of a wide range of neurotransmitters (Hanson et al. 1997; Rizo and Südhof 2012). Genetic studies in mouse models and human populations have suggested that alterations of SNAP-25 gene expression or impaired function may contribute to distinct neuropsychiatric and neurological disorders, e.g., attention-deficit/hyperactivity disorder, schizophrenia or epilepsy (Corradini et al. 2009; Guerini et al. 2011), and SNAP-25 regulates voltage-gated calcium channels thereby modulating presynaptic calcium concentration in the CNS (Catterall 1999; Verderio et al. 2004; Zamponi 2003).
For the human ENS, there are only few data available concerning the expression levels and distribution pattern of SNAP-25. Nirasawa et al. (2001) have studied SNAP-25 distribution in normal and aganglionic colonic segments obtained from infant patients with Hirschsprung’s disease (HSCR). In these infant specimens, immunohistochemical staining pattern of SNAP-25 was similar to those observed in the present study using adult specimens (e.g., immunoreactive signals in intramuscular nerve fibers, neuropil, neuronal somata). In addition, by using co-localization studies with the pan-neuronal marker PGP-9.5 (Krammer et al. 1993), we could further confirm that SNAP-25 localization is confined to enteric neurons and exhibits a distinct granular staining pattern. Beckett et al. (2005) detected transcripts for the presynaptic proteins synaptotagmin, syntaxin and SNAP-25 in the murine tunica muscularis of the stomach, whereas we analyzed the site-specific gene expression of SNAP-25 in different colonic layers and found highest SNAP-25 mRNA expression in myenteric ganglia.
The abundant presence of SNAP-25 both at gene and protein level in the ENS suggests a crucial role for SNAP-25 in neurotransmitter release not only in the CNS but also in the GI tract. Since also other components of the exocytosis machinery have been demonstrated in the ENS, e.g., the v-SNARE synaptobrevin and the synaptophysin (Böttner et al. 2013b; Sharrad et al. 2013) or syntaxin 1 (Aguado et al. 1999; Nirasawa et al. 1997), it is likely that the t-SNARE SNAP-25 fulfills similar functions in the ENS as described for the CNS.
At the level of the CNS, only recently it could be shown that SNAP-25 is able to modulate responsiveness to selected neurotransmitter systems. Antonucci et al. (2013) revealed a selective enhancement of glutamatergic but not γ-aminobutyric acid (GABA)-ergic neurotransmission as a result of reduced expression of SNAP-25 in hippocampal cultures. This function might relate to the ability of SNAP-25 to modulate voltage-gated calcium channels and thus presynaptic calcium ion concentration in the CNS (Condliffe et al. 2010). Since enteric neurons are also known to respond to both glutamate (Giaroni et al. 2003; Kirchgessner 2001) and GABA (Hyland and Cryan 2010), it is suggestive that SNAP-25 acts in a similar manner in the ENS.
Analysis of the normal expression and distribution pattern of SNAP-25 in healthy human intestine is of special importance, because these data provide a basis to reliably evaluate putative alterations of SNAP-25 under pathological conditions. Evidence for relevant SNAP-25 changes in functional bowel diseases is given by our observation of decreased SNAP-25 mRNA expression and immunoreactivity in patients with DD. SNAP-25 is actively involved in the neurotransmitter release machinery, and enteric neurotransmitter imbalance is associated with a broad range of intestinal motility disorders, e.g., slow-transit constipation, gastroparesis, chronic intestinal pseudoobstruction, IBS (Di Nardo et al. 2008; Knowles et al. 2010). Thus, the detailed characterization of SNAP-25 at the level of the ENS might be a promising tool to detect further structural alterations (e.g., impaired synaptic vesicle apparatus) underlying the aforementioned functional bowel diseases.
SNAP-25 and synaptic varicosities
The granular immunohistochemical staining pattern of SNAP-25 observed in the human ENS was reproducible in the in vitro model of cultured rat enteric neurons. The granula were localized both within ganglia and interganglionic nerve fibers of colonic nerve plexus as well as in enteric nerve cultures as demonstrated by co-localization of SNAP-25 with the pan-neuronal marker PGP 9.5. Scanning electron microscopical evaluation of the nervous meshwork formed by cultured enteric nerve cells showed several axonal swellings along nerve fibers and nerve cell aggregates resembling typical morphological features of synaptic varicosities. Ultrastructural analysis of these nerve fiber protrusions revealed the presence of densely packed synaptic vesicles most likely corresponding to the SNAP-25 immunoreactive granula observed at lightmicroscopical level. The synaptic vesicles exhibited different sizes and variable electron densities typically observed in enteric synaptic varicosities (Burnstock 2008). Low-dense core vesicles corresponded to small synaptic vesicles, whereas the larger vesicles constituted large-dense core vesicles containing different types of neurotransmitters (Bruns and Jahn 1995). These ultrastructural findings give evidence that GDNF-stimulated enteric nerve cell cultures develop synaptic varicosities with similar structural features observed in the human intestine including the granular staining pattern of the synaptic vesicle protein SNAP-25.
Induction of SNAP-25 expression by neurotrophic factors
In the ENS, the role of neurotrophic factors such as GDNF for the mediation of synaptic plasticity by regulating components of the enteric synaptic vesicle apparatus as shown for the CNS (Lo 1995; McAllister et al. 1999; Wang et al. 2003) remains unclear. To investigate the regulatory effect of GDNF on the expression of SNAP-25, we used an in vitro model of cultured enteric neurons. This cell culture model was previously described by Schäfer et al. and could demonstrate an increased survival and differentiation of enteric neurons after GDNF treatment (Böttner et al. 2013a; Schäfer and Mestres 1999; Schäfer et al. 1997). We analyzed the mRNA expression of the t-SNARE snap-25 by RT-qPCR in cultured enteric neurons and found a dose-dependent increase in snap-25 mRNA expression upon GDNF treatment. This finding completes our previous study in which gene expression levels of the v-SNARE synaptobrevin and also the v-SNARE-associated protein synaptophysin were upregulated by GDNF treatment (Böttner et al. 2013b) and further strengthens the role of GDNF to promote synaptogenesis in general and neural plasticity in the ENS.
This concept is in line with a report from Zeng et al. who demonstrated that GDNF enhanced synaptic communication via suppression of voltage-gated K+ (Kv) channel activity (Kv 2.1) in cultured myenteric neurons (Zeng et al. 2009). Interestingly, both activation and inactivation of Kv 2.1 are affected, depending on the assembly/disassembly of the binary complex SNAP-25/syntaxin 1A in neuroendocrine cells (Michaelevski et al. 2003; Tsuk et al. 2008) pointing to a possible role of neurotrophic factors in regulating synaptic communication via direct interaction of t-SNARE proteins with potassium channels.
Moreover, the data suggest that a decreased expression of neurotrophic factors such as GDNF may ultimately lead to impaired enteric neurotransmission. Among the spectrum of intestinal innervation disorders, an altered GDNF system has been demonstrated not only for HSCR (Butler Tjaden and Trainor 2013) but also for DD (Böttner et al. 2013a). In fact, we could reveal a decreased density and expression level of SNAP-25 in patients with DD, speaking in favor of the link between an impaired GDNF system and an altered synaptic vesicle. Based on these data, it is intriguing to speculate whether other enteric neuropathies are also characterized by a deficiency of the GDNF system affecting the composition of the synaptic vesicle apparatus and thereby impairing an appropriate neurotransmitter release and transmission.
Conclusion
In summary, the present study has shown abundant expression of SNAP-25 as major component of the neurotransmitter release machinery at the level of the human ENS. As upregulation of SNAP-25 is induced by the neurotrophic factor GDNF, both decreased levels of SNAP-25 and an impaired GDNF system might compromise enteric neurotransmission contributing to the pathogenesis of intestinal motility disorders. Future studies have to elucidate whether SNAP-25 and other synaptic vesicle markers may be promising candidate molecules to further characterize human enteric neuropathies and serve as histopathological diagnostic tools.
Abbreviations
- CNS:
-
Central nervous system
- DD:
-
Diverticular disease
- ENS:
-
Enteric nervous system
- GABA:
-
γ-Aminobutyric acid
- GDNF:
-
Glial cell line-derived neurotropic factor
- GFRα1:
-
GDNF family receptor alpha 1
- GI:
-
Gastrointestinal
- HSCR:
-
Hirschsprung’s disease
- IBS:
-
Irritable bowel syndrome
- LMD:
-
Laser capture microdissection
- qPCR:
-
Quantitative PCR
- RET:
-
Rearranged during transfection
- SNAP-25:
-
Synaptosome-associated protein 25 kDa
- SNARE:
-
Soluble N-ethylmaleimide-sensitive factor attachment receptor
References
Aguado F, Majo G, Ruiz-Montasell B, Llorens J, Marsal J, Blasi J (1999) Syntaxin 1A and 1B display distinct distribution patterns in the rat peripheral nervous system. Neuroscience 88:437–446
Airaksinen MS, Saarma M (2002) The GDNF family: signalling, biological functions and therapeutic value. Nat Rev Neurosci 3:383–394
Antonucci F, Corradini I, Morini R, Fossati G, Menna E, Pozzi D, Pacioni S, Verderio C, Bacci A, Matteoli M (2013) Reduced SNAP-25 alters short-term plasticity at developing glutamatergic synapses. EMBO Rep 14:645–651
Beckett EA, Takeda Y, Yanase H, Sanders KM, Ward SM (2005) Synaptic specializations exist between enteric motor nerves and interstitial cells of Cajal in the murine stomach. J Comp Neurol 493:193–206
Benarroch EE (2013) Synaptic vesicle exocytosis: molecular mechanisms and clinical implications. Neurology 80:1981–1988
Bottner M, Bar F, Von Koschitzky H, Tafazzoli K, Roblick UJ, Bruch HP, Wedel T (2010) Laser microdissection as a new tool to investigate site-specific gene expression in enteric ganglia of the human intestine. Neurogastroenterol Motil 22(168–172):e152
Böttner M, Bar F, Von Koschitzky H, Tafazzoli K, Roblick UJ, Bruch HP, Wedel T (2010) Laser microdissection as a new tool to investigate site-specific gene expression in enteric ganglia of the human intestine. Neurogastroenterol Motil 22(168–172):e152
Böttner M, Zorenkov D, Hellwig I, Barrenschee M, Harde J, Fricke T, Deuschl G, Egberts JH, Becker T, Fritscher-Ravens A, Arlt A, Wedel T (2012) Expression pattern and localization of alpha-synuclein in the human enteric nervous system. Neurobiology Dis 48:474–480
Böttner M, Barrenschee M, Hellwig I, Harde J, Egberts JH, Becker T, Zorenkov D, Schäfer KH, Wedel T (2013a) The GDNF system is altered in diverticular disease—implications for pathogenesis. PLoS One 8:e66290
Böttner M, Harde J, Barrenschee M, Hellwig I, Vogel I, Ebsen M, Wedel T (2013b) GDNF induces synaptic vesicle markers in enteric neurons. Neurosci Res 77:128–136
Bruns D, Jahn R (1995) Real-time measurement of transmitter release from single synaptic vesicles. Nature 377:62–65
Burgoyne RD, Morgan A (2011) Chaperoning the SNAREs: a role in preventing neurodegeneration? Nat Cell Biol 13:8–9
Burnstock G (2008) Non-synaptic transmission at autonomic neuroeffector junctions. Neurochem Int 52:14–25
Butler Tjaden NE, Trainor PA (2013) The developmental etiology and pathogenesis of Hirschsprung disease. Transl Res 162:1–15
Catterall WA (1999) Interactions of presynaptic Ca2+ channels and snare proteins in neurotransmitter release. Ann NY Acad Sci 868:144–159
Condliffe SB, Corradini I, Pozzi D, Verderio C, Matteoli M (2010) Endogenous SNAP-25 regulates native voltage-gated calcium channels in glutamatergic neurons. J Biol Chem 285:24968–24976
Corradini I, Verderio C, Sala M, Wilson MC, Matteoli M (2009) SNAP-25 in neuropsychiatric disorders. Ann NY Acad Sci 1152:93–99
Costa M, Brookes SJ (1994) The enteric nervous system. Am J Gastroenterol 89:S129–S137
Costa M, Brookes SJ, Hennig GW (2000) Anatomy and physiology of the enteric nervous system. Gut 47(Suppl 4):iv15–iv19 discussion iv26
Di Nardo G, Blandizzi C, Volta U, Colucci R, Stanghellini V, Barbara G, Del Tacca M, Tonini M, Corinaldesi R, De Giorgio R (2008) Review article: molecular, pathological and therapeutic features of human enteric neuropathies. Aliment Pharmacol Ther 28:25–42
Furness JB (2006) The enteric nervous system. Blackwell, Oxford
Furness JB (2012) The enteric nervous system and neurogastroenterology. Nat Rev Gastroenterol Hepatol 9:286–294
Giaroni C, Zanetti E, Chiaravalli AM, Albarello L, Dominioni L, Capella C, Lecchini S, Frigo G (2003) Evidence for a glutamatergic modulation of the cholinergic function in the human enteric nervous system via NMDA receptors. Eur J Pharmacol 476:63–69
Guerini FR, Bolognesi E, Chiappedi M, Manca S, Ghezzo A, Agliardi C, Sotgiu S, Usai S, Matteoli M, Clerici M (2011) SNAP-25 single nucleotide polymorphisms are associated with hyperactivity in autism spectrum disorders. Pharmacol Res 64:283–288
Hanson PI, Heuser JE, Jahn R (1997) Neurotransmitter release—four years of SNARE complexes. Curr Opin Neurobiol 7:310–315
Hyland NP, Cryan JF (2010) A Gut Feeling about GABA: focus on GABA(B) Receptors. Front Pharmacol 1:124
Jahn R, Scheller RH (2006) SNAREs—engines for membrane fusion. Nat Rev Mol Cell Biol 7:631–643
Kirchgessner AL (2001) Glutamate in the enteric nervous system. Curr Opin Pharmacol 1:591–596
Knowles CH, De Giorgio R, Kapur RP, Bruder E, Farrugia G, Geboes K, Gershon MD, Hutson J, Lindberg G, Martin JE, Meier-Ruge WA, Milla PJ, Smith VV, Vandervinden JM, Veress B, Wedel T (2009) Gastrointestinal neuromuscular pathology: guidelines for histological techniques and reporting on behalf of the Gastro 2009 International Working Group. Acta Neuropathol 118:271–301
Knowles CH, De Giorgio R, Kapur RP, Bruder E, Farrugia G, Geboes K, Lindberg G, Martin JE, Meier-Ruge WA, Milla PJ, Smith VV, Vandervinden JM, Veress B, Wedel T (2010) The London Classification of gastrointestinal neuromuscular pathology: report on behalf of the Gastro 2009 International Working Group. Gut 59:882–887
Krammer HJ, Karahan ST, Rumpel E, Klinger M, Kuhnel W (1993) Immunohistochemical visualization of the enteric nervous system using antibodies against protein gene product (PGP) 9.5. Ann Anat 175:321–325
Ledda F, Paratcha G, Sandoval-Guzman T, Ibanez CF (2007) GDNF and GFRalpha1 promote formation of neuronal synapses by ligand-induced cell adhesion. Nat Neurosci 10:293–300
Lin LF, Doherty DH, Lile JD, Bektesh S, Collins F (1993) GDNF: a glial cell line-derived neurotrophic factor for midbrain dopaminergic neurons. Science 260:1130–1132
Lo DC (1995) Neurotrophic factors and synaptic plasticity. Neuron 15:979–981
Lourenssen S, Houpt ER, Chadee K, Blennerhassett MG (2010) Entamoeba histolytica infection and secreted proteins proteolytically damage enteric neurons. Infect Immun 78:5332–5340
McAllister AK, Katz LC, Lo DC (1999) Neurotrophins and synaptic plasticity. Annu Rev Neurosci 22:295–318
Michaelevski I, Chikvashvili D, Tsuk S, Singer-Lahat D, Kang Y, Linial M, Gaisano HY, Fili O, Lotan I (2003) Direct interaction of target SNAREs with the Kv2.1 channel. Modal regulation of channel activation and inactivation gating. J Biol Chem 278:34320–34330
Nirasawa Y, Ito Y, Seki N, Akagawa K (1997) HPC-1/syntaxin-1A activity in the enteric nervous system of developing rat gastrointestinal tract. J Smooth Muscle Res 33:61–66
Nirasawa Y, Ito Y, Fujiwara T, Seki N, Tanaka H, Akagawa K (2001) Altered immunoreactivity of HPC-1/syntaxin 1A in proliferated nerve fibers in the human aganglionic colon of Hirschsprung’s disease. J Mol Neurosci 16:13–19
Poli E, Lazzaretti M, Grandi D, Pozzoli C, Coruzzi G (2001) Morphological and functional alterations of the myenteric plexus in rats with TNBS-induced colitis. Neurochem Res 26:1085–1093
R-Core-Team (2012) R: a language and environment for statistical computing. R Foundation for Statistical Computing, Vienna
Rizo J, Südhof TC (2002) Snares and Munc18 in synaptic vesicle fusion. Nat Rev Neurosci 3:641–653
Rizo J, Südhof TC (2012) The membrane fusion enigma: SNAREs, Sec1/Munc18 proteins, and their accomplices—guilty as charged? Annu Rev Cell Dev Biol 28:279–308
Rodrigues DM, Li AY, Nair DG, Blennerhassett MG (2011) Glial cell line-derived neurotrophic factor is a key neurotrophin in the postnatal enteric nervous system. Neurogastroenterol Motil 23:e44–e56
Schäfer KH, Mestres P (1999) The GDNF-induced neurite outgrowth and neuronal survival in dissociated myenteric plexus cultures of the rat small intestine decreases postnatally. Exp Brain Res 125:447–452
Schäfer KH, Saffrey MJ, Burnstock G, Mestres-Ventura P (1997) A new method for the isolation of myenteric plexus from the newborn rat gastrointestinal tract. Brain Res Brain Res Protoc 1:109–113
Sharma M, Burre J, Bronk P, Zhang Y, Xu W, Südhof TC (2012) CSPalpha knockout causes neurodegeneration by impairing SNAP-25 function. EMBO J 31:829–841
Sharrad DF, Gai WP, Brookes SJ (2013) Selective coexpression of synaptic proteins, alpha-synuclein, cysteine string protein-alpha, synaptophysin, synaptotagmin-1, and synaptobrevin-2 in vesicular acetylcholine transporter-immunoreactive axons in the guinea pig ileum. J Comp Neurol 521:2523–2537
Snoek SA, Verstege MI, Boeckxstaens GE, van den Wijngaard RM, de Jonge WJ (2010) The enteric nervous system as a regulator of intestinal epithelial barrier function in health and disease. Expert Rev Gastroenterol Hepatol 4:637–651
Südhof TC (1995) The synaptic vesicle cycle: a cascade of protein–protein interactions. Nature 375:645–653
Südhof TC (2004) The synaptic vesicle cycle. Annu Rev Neurosci 27:509–547
Südhof TC, Rizo J (2011) Synaptic vesicle exocytosis. Cold Spring Harb Perspect Biol 3:a005637
Tsuk S, Lvov A, Michaelevski I, Chikvashvili D, Lotan I (2008) Formation of the full SNARE complex eliminates interactions of its individual protein components with the Kv2.1 channel. Biochemistry 47:8342–8349
Verderio C, Pozzi D, Pravettoni E, Inverardi F, Schenk U, Coco S, Proux-Gillardeaux V, Galli T, Rossetto O, Frassoni C, Matteoli M (2004) SNAP-25 modulation of calcium dynamics underlies differences in GABAergic and glutamatergic responsiveness to depolarization. Neuron 41:599–610
von Boyen GB, Reinshagen M, Steinkamp M, Adler G, Kirsch J (2002) Enteric nervous plasticity and development: dependence on neurotrophic factors. J Gastroenterol 37:583–588
Wang J, Chen G, Lu B, Wu CP (2003) GDNF acutely potentiates Ca2+ channels and excitatory synaptic transmission in midbrain dopaminergic neurons. Neurosignals 12:78–88
Wedel T, Böttner M (2014) Anatomy and pathogenesis of diverticular disease. Chirurg 85:281–288
Wedel T, Büsing V, Heinrichs G, Nohroudi K, Bruch HP, Roblick U, Böttner M (2010) Diverticular disease is associated with an enteric neuropathy as revealed by morphometric analysis. Neurogastroenterol Motil 22:407–414
Zamponi GW (2003) Regulation of presynaptic calcium channels by synaptic proteins. J Pharmacol Sci 92:79–83
Zeng F, Watson RP, Nash MS (2009) Glial cell-derived neurotrophic factor enhances synaptic communication and 5-hydroxytryptamine 3a receptor expression in enteric neurons. Gastroenterology 138:1491–1501
Acknowledgments
The authors thank Karin Stengel, Inka Geurink, Miriam Lemmer, Frank Lichte, Bettina Facompré and Clemens Franke (Institute of Anatomy, Christian-Albrechts-University of Kiel) for their excellent technical assistance. This work was supported by research grants from the German Research Foundation (Deutsche Forschungsgemeinschaft, DFG WE 2366/4-3) and the Faculty of Medicine, University of Kiel (F347022). The funding sources have no role in study design, management of data and writing of the paper.
Conflict of interest
The authors disclose no competing interests.
Author information
Authors and Affiliations
Corresponding author
Additional information
M. Barrenschee and M. Böttner have contributed equally to this work.
Rights and permissions
About this article
Cite this article
Barrenschee, M., Böttner, M., Harde, J. et al. SNAP-25 is abundantly expressed in enteric neuronal networks and upregulated by the neurotrophic factor GDNF. Histochem Cell Biol 143, 611–623 (2015). https://doi.org/10.1007/s00418-015-1310-x
Accepted:
Published:
Issue Date:
DOI: https://doi.org/10.1007/s00418-015-1310-x