Abstract
Purpose
Resistance exercise and amino acid availability are positive regulators of muscle protein net balance (NB). However, anabolic responses to resistance exercise and protein supplementation deserve further elucidation. The purpose was to compare intakes of whey, caseinate (both: 0.30 g/kg lean body mass), or a non-caloric control after heavy resistance exercise on protein turnover and mRNA expressions of forkhead homeobox type O (FOXO) isoforms, muscle RING finger 1 (MuRF1), and Atrogin1 in young healthy males.
Methods
Protein turnover was determined by stable isotope-labeled leucine and femoral arteriovenous blood samples at rest and during 6-h recovery. Muscle biopsies were collected at −60 min (rest) and at 60, 210, and 360 min in the recovery period.
Results
During recovery, leucine NB was significantly higher in the protein groups compared to control (P < 0.001). Differences in leucine NB, rate of disappearance, and oxidation were observed in the early recovery period between whey and caseinate. FOXO1A and MuRF1 were upregulated at 60 and 210 min, and, in contrast, FOXO3 and Atrogin1 were downregulated at 210 and 360 min. For leucine rate of appearance and all FOXO and atrogene mRNA expressions, no differences were observed between groups.
Conclusions
Whey and caseinate were equally superior to control in the 6-h recovery period and displayed temporal differences with whey having a fast and superior effect in the early part of the recovery period. Effects on mRNA expressions indicate different regulatory mechanisms on the ubiquitin ligases MuRF1 and Atrogin1 in recovery from heavy resistance exercise.
Similar content being viewed by others
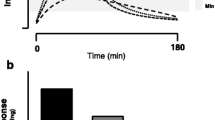
Avoid common mistakes on your manuscript.
Background
Exercise and nutrition have profound effects on muscle protein turnover and markedly affect muscle protein balance determined by the balance between muscle protein synthesis (MPS) and muscle protein breakdown (MPB) [1–3]. In particular, resistance exercise has profound effects both measured acutely in the hours after the performance [3–6], but also in terms of muscle hypertrophy over longitudinal training regimes [7, 8]. Furthermore, protein or amino acid (AA) ingestion in relation to resistance exercise has been documented to have additive effects on the anabolic response [1, 9, 10].
Bovine milk proteins serve as a biologically high-quality AA source with a complete profile of essential amino acids (EAA) [11]. However, the two major protein fractions in milk, whey and casein, display different digestion and absorption rates with whey being rapidly and casein slowly digested and hence absorbed [12, 13]. These different digestive properties have been shown to affect whole-body protein turnover and AA retention differently [12, 14].
In relation to resistance exercise, few attempts have been done to determine the effects of and possible differences between whey and micellar casein/caseinate on muscle specific protein turnover, and the results are not consistent [15–20]. We have previously shown that whey and caseinate supplementation ingested immediately after completion of heavy resistance exercise resulted in an overall equal response in fractional synthesis rate (FSR) of myofibrillar proteins in the 1- to 6-h recovery period [17]. This result was observed together with temporal differences in insulin and AA concentrations, and there was a tendency toward a temporal shift in the FSR response between whey and caseinate from the early part (1–3.5 h) of the recovery period compared to the late part (3.5–6 h).
Muscle protein turnover regulation is in part mediated through downstream signaling of the insulin-like growth factor I (IGF-I)/insulin receptor affecting both MPS and MPB via v-akt murine thymoma viral oncogene (Akt) [21, 22]. IGF-I is capable of decreasing protein breakdown by activating the phosphatidylinositol 3-kinase/Akt pathway phosphorylating and inhibiting the function of the forkhead homeobox type O (FOXO) family of transcription factors, thereby regulating the expression of the atrogenes, Atrogin1 and muscle RING finger 1 (MuRF1), which are central mediators in the ubiquitin–proteasome system [23, 24]. Different models of muscle atrophy including fasting, denervation, and immobilization have documented that gene expression of MuRF1 and Atrogin1 is upregulated in these catabolic states [25–29]. In relation to exercise, MuRF1 mRNA expression has been shown to increase acutely following one session of heavy resistance exercise [30–32], although one study reported unaffected gene expression [33]. In contrast, results indicate that Atrogin1 mRNA is decreased or unchanged acutely following exercise [30–32].
The aim of the present study was to investigate whether intakes of whey, caseinate, or a non-caloric control drink after a single bout of heavy resistance exercise affect muscle protein turnover measured with a leucine stable isotope tracer model in young, moderately active males. Furthermore, changes in mRNA expressions of IGF-I and FOXO isoforms, MuRF1, and Atrogin1 were investigated. We hypothesized that whey and caseinate would have superior effects on muscle protein turnover and gene expressions after performance of heavy resistance exercise as compared to the non-caloric control drink and that whey protein would induce a rapid but transient response, whereas caseinate would result in a moderate and more persistent response.
Methods
Subjects
Healthy and moderately active young men with no history of regular participation in endurance or resistance training within the last 6 months were recruited (Table 1). A large part of the participants were the same individuals as studied in our previous study [17]; however, due to subject retention and methodological constraints, the group compositions in the present data set are different. All participants were non-smokers and on a normal Western diet adequate with regard to protein content (>0.8 g/kg/day). Study design, purpose, and possible risks were explained to each subject, who subsequently gave written consent to participate in the project, which were adhering to the Helsinki declaration and approved by the Ethics Committee of Copenhagen and Frederiksberg (H-KF2007-0014).
Experimental protocol
Subjects had their lean body mass (LBM) determined by DXA scanning (Lunar DPX-IQ, GE Healthcare, Chalfont St. Giles, UK) and had their 1 repetition maximum (1RM) leg-extension strength determined at least 2 weeks before the experiment. Subjects refrained from strenuous physical activity during the three pre-experiment days. The experiment was designed as an acute exercise trial with pre-exercise baseline measurements followed by post-exercise/drink measurements during 6 h (Fig. 1). Subjects arrived to the laboratory by taxi at 0700 hours after an overnight fast. Antecubital catheters were inserted and isotopic background blood and breathe CO2 samples obtained.
Human experiment protocol. L-[1-13C]leucine was infused during 9 h while a single bout of heavy resistance exercise was performed with subsequent intake of the study drink. The study drinks consisted of L-[1-13C]leucine-labeled whey or caseinate, or a non-caloric control drink. Arteriovenous (A-V) blood samples were collected throughout the protocol, and a total of four muscle biopsies were obtained in the vastus lateralis of the exercise leg
Stable isotope infusion
At time −3 h (0 h denotes end of exercise), the primed (15 μmol/kg L-[1-13C]leucine and 2.5 μmol/kg Na H13CO3) continuous (15 μmol/kg/h L-[1-13C]leucine) intravenous infusion of tracers (Cambridge Isotope Laboratories, Andover, MA, USA) was started. This infusion design allowed 2 h for free leucine tracer in blood and muscle to equilibrate before any physiological measurements were started.
Femoral arteriovenous blood and muscle biopsy sampling
After start of tracer infusion, the femoral artery and vein of the exercise leg were cannulated under local anesthesia (lidocaine, 1 %). Applying the Seldinger technique, 20 gauge catheters (Arrow, ES-04150, Reading, PA) were inserted. Arteriovenous blood samples were obtained by first collecting the arterial and secondly, the venous blood. Blood was centrifuged 10 min at 3,060×g at 4 °C (Eppendorf Centrifuge 5810R, Hamburg, Germany), and plasma was collected and stored at −80 °C.
Muscle biopsies from vastus lateralis were taken under local anesthesia (lidocaine, 1 %) with 4-mm Bergström needles (Stille, Stockholm, Sweden) applying manual suction. Blood, visible fat, and connective tissue were quickly removed from the specimens, which subsequently were frozen in liquid nitrogen and stored at −80 °C.
Exercise protocol
The resistance exercise protocol consisted of 10 sets, separated by 3-min rest, consisting of 8 repetitions at 80 % of 1RM, which aimed to stimulate the quadriceps muscle maximally. The exercise was one-legged, seated leg-extension (Technogym, Super Executive Line, Gambottola, Italy) with a range of motion from 100 to 30° (0° denotes a fully extended leg).
Protein supplementation
The drink containing either [1-13C]leucine-whey, [1-13C]leucine-caseinate (in the form of calcium caseinate), or a non-caloric water drink was given immediately after exercise. The labeled milk proteins were produced by infusing lactating cows with L-[1-13C]leucine and collecting the enriched milk as described in detail elsewhere [17]. By having the proteins labeled (10.0 % tracer-to-trace ratio (TTR)), we aimed to avoid a significant dilution of our leucine tracer in the recovery period. All subjects were blinded with regard to what drink they received. The amount of protein given was 0.30 g/kg LBM corresponding to 17–18 g, which was dissolved into 400 mL water and consumed within 5 min. The AA content of the milk proteins are displayed in Supplementary Table 1.
Leg blood flow
Leg blood flow (LBF) measurements were done in the femoral artery and carried out on a separate occasion but under similar conditions as on the experiment day. Before LBF measurements, subjects had rested in a supine position for 1 h. LBF was estimated using ultrasound Doppler techniques (LOGIQ 9, GE Healthcare, Chalfont St. Giles, UK) [34] as LBF (mL/min) = V mean(m/s)·A(m2)·60 × 106. V mean is mean blood velocity, and by assuming a circular femoral artery, A is the cross-sectional area of the artery (A(m2) = π·r 2). LBF were correlated to LBM of the leg (LBMleg), and values were expressed in mL min−1 100 g LBM −1leg . While there is no substantial AA transport via blood cells, calculations of leucine kinetics were based on plasma flow, which was derived from LBF by use of the hematocrit.
Local leg leucine oxidation
Femoral arterial and venous blood samples were collected anaerobically into pre-heparinized syringes (PICO50, Radiometer, Copenhagen, Denmark) and thoroughly mixed. Seventy microliters was injected into a blood gas analyzing system (ABL500) and 35 μL, into a hemoxymeter system (OSM3) (both Radiometer, Copenhagen, Denmark) for analysis of partial pressure of CO2, blood pH, O2 saturation, and hemoglobin. For determination of 13CO2 enrichment in blood, 500 μL blood was transferred to a 10 mL vacutainer (BD Vacutainers, Franklin Lakes, NJ, USA), and subsequently, 500 μL of 2.5 mol/L phosphoric acid was added to liberate CO2 into the headspace of the vacutainer. The remaining vacuum was eliminated with helium. The 13CO2/12CO2 ratio was determined by gas chromatography–isotope ratio mass spectrometry (Deltaplus XL, Thermo Finnigan, Bremen, Germany). The isotopic abundance of blood-derived 13CO2 was calculated from the δ ‰ difference between 13C/12C of the sample and a known laboratory reference standard related to Pee Dee Belemnitella limestone.
Arteriovenous enrichments and concentrations
Arteriovenous plasma leucine enrichments and concentrations were determined by gas chromatography-mass spectrometry (GC–MS) with use of the internal tracer standard method [35]. 150 μL plasma, 200 μL internal standard (60 μmol/L L-[13C6]leucine (Cambridge Isotope Laboratories, Andover, MA, USA)), and 1 mL acetic acid were thoroughly mixed. AA was purified over cation exchange resin (Dovex AG-50 W, Bio-Rad, Copenhagen, Denmark) columns and the AA derivatized using N-tert-butyldimethylsilyl-N-methyltrifluoroacetamide with 1 % tert-butyldimethylchlorosilane (Sigma-Aldrich, St. Louis, MO). The derivatized sample was injected in the programmed-temperature vaporization mode and carried by a constant helium flow (1.8 mL/min) into the GC–MS system (GC: Trace GC 2000 series, Thermo Quest Finnigan, Paris, France; MS: Automass Multi, Thermo Quest Finnigan, Paris, France) and separated by a capillary column (CP-Sil 8 CB low bleed 30 m 0.32 mm, coating 0.25 μm, ChromPack, Varian, Palo Alto, CA, USA) measuring leucine fragment ions M (m/z 302), M+1 (m/z 303), and M+6 (m/z 308).
Calculations
Leucine kinetics were calculated using the 2-compartment model approach [36–39], which provides information regarding the leucine kinetics across the leg. Leucine kinetics together with local leg leucine oxidation gives the non-oxidative leucine disposal (NOLD), which is an estimate of protein synthesis [39].
Each kinetic parameter is defined as follows [36, 37, 39]:
where C a and C v are the arterial and venous plasma concentrations, respectively, and E a and E v are the arterial and venous enrichments (TTR), respectively.
The local leg leucine oxidation was calculated as [39]:
where [13CO2]v and [13CO2]a is 13CO2 enrichment multiplied by CO2 concentration in the venous and arterial blood, respectively, c is the bicarbonate retention factor (0.8) [40–42], [Hb] is hemoglobin concentration of the blood, sO2 is O2 saturation, 2.226 is the conversion factor from mmol/L to mL/100 mL, s and pK! are the plasma solubility coefficient and apparent pK! of the bicarbonate system, respectively. Standard values of s and pK! at 37 °C and pH 7.4 are 0.0307 and 6.0907. pCO2 is partial pressure of CO2.
Area under the curve (AUC) was calculated from 30 to 360 min. Since leucine is oxidized in muscle, the leucine NB AUC was subtracted from the leucine oxidation AUC to give a final measure of net NOLD within the leg. All kinetic parameters are expressed as nmol min−1 100 g LBM −1leg .
Muscle mRNA measurements
The amount of mRNA for IGF-IEa, IGF-IEb, IGF-IEc, FOXO1A, FOXO3, FOXO4, MuRF1, and Atrogin1 was measured using real-time RT-PCR. Approximately 10 mg of muscle tissue was homogenized in 1,000 μL TriReagent (RT 118, Molecular Research Center, Cincinnati, OH) using a FastPrep-24 (MP Biomedicals, Solon, OH), with muscle tissue placed in a 2-mL microvial with a screw cap and 1 siliciumcarbide crystal and 5 0.4-mm steel beads (Biospec Products, Bartlesville, OK, USA). Hereafter, 100 μL bromo-chloropropane (Molecular Research Center, Cincinnati, OH, USA) was added to the homogenized sample to separate the sample into an aqueous and organic phase. The RNA was precipitated from the aqueous phase using isopropanol and hereafter washed with ethanol and dissolved in RNase-free water. The RNA was then reprecipitated using NaAc, washed in ethanol and dissolved in RNase-free water. RNA concentration was determined by spectroscopy, and RNA quality was ensured by gel electrophoresis.
Synthesis of cDNA was performed using Omniscript reverse transcriptase (Qiagen, Hilden, Germany). The cDNA synthesis was performed in 20 μL on 500 ng of muscle RNA. Hereafter, the cDNA was diluted 20 times in TE-buffer containing Salmon DNA (10 mmol/L Tris–HCl, 1 mmol/L EDTA, 1 ng/mL Salmon sperm DNA, pH 8). For each target, 5 μL of diluted cDNA was amplified in a total of 25 μL of Quantitect SYBR Green Master Mix (Qiagen) with specific primers (0.1 μmol/L each, Supplementary Table 2) on a real-time PCR machine (Stratagene Mx3005P, Agilent Technologies, Santa Clara, CA). The thermal profile was set to 95 °C for 10 min → (95 °C for 15 s → 58 °C for 30 s → 63 °C for 90 s) × 50 → 95 °C for 60 s → 55 °C for 30 s → 95 °C for 30 s. Signal intensity was measured at the 63 °C step, and the threshold cycle (C t) values were related to a standard curve made from the cloned PCR product. Specificity of the PCR product was confirmed by melting curve analysis after amplification (the last step from 55 to 95 °C). Glyceralaldehyde-3-phosphate dehydrogenase (GAPDH) was used as reference gene. We measured both GAPDH and ribosomal protein large P0 (RPLP0) and found that RPLP0 when normalized to GAPDH showed significant effects of time (see “Results” section). This could be due to an actual increase in RPLP0, or it could be due to a decrease in the normalization gene, GAPDH. However, we do not suspect an enzyme in the glycolysis to be downregulated as a consequence of our intervention, and, on the other hand, it seems more plausible that RPLP0 could be moderately increased. Therefore, GAPDH was considered to be most appropriate as reference gene. Furthermore, the magnitude and divergent directions of the measured mRNA targets showed no signs of a systematic normalization bias when normalized to GAPDH.
Statistics
Statistical analyses were carried out as 2-factor (group and time) ANOVA with group as non-repeated and time as repeated measures. Since the whey and caseinate groups consist of paired and unpaired legs, we have chosen to do unpaired statistics between all three groups. Student–Newman–Keuls post hoc tests were performed to assess specific differences between groups and time points. The subject characteristics and AUC responses between groups were analyzed with 1-factor non-repeated measure ANOVA. All values are presented as mean ± SE. The mRNA data were log-transformed before statistical analyses to avoid skewed distribution and are presented as geometric means ± back-transformed SE. Level of significance was set at P < 0.05, and analyses were performed with SigmaPlot 11.0 (Systat Software Inc., San Jose, CA, USA).
Results
Subject characteristics
There were no significant differences between groups in any of the subject characteristics (Table 1). Mean LBF values were not significantly different, and group averages were 2.40 ± 0.16, 2.26 ± 0.19, and 2.77 ± 0.24 mL min−1 100 g LBM −1leg for the whey, caseinate, and control group, respectively.
Leucine enrichments
In general, the leucine enrichments in the arteriovenous compartments were relatively stable (Fig. 2). However, in the arterial compartment, a significant interaction was observed (P < 0.01), and within the whey group, the enrichment at 30 min was significant lower than 90–360 min (P < 0.05; Fig. 2a) and the enrichment at 60 min was significant lower than 180 and 300–360 min (P < 0.05). Within the caseinate group, the enrichment at 30 min was significant lower than 180–240 min (P < 0.05; Fig. 2b). In the control group, no effects of time were observed (Fig. 2c). Group differences were only observed at −60 min with caseinate being higher than whey and control (P < 0.05). In the venous compartment, there was a main effect of group (P < 0.05) and time (P < 0.001). The caseinate group had significantly higher enrichments than control (P < 0.05), and time −60 min was significantly lower than all other time points (P < 0.05). Furthermore, 30 min was lower than 300 min (P < 0.05).
Leg plasma [1-13C]leucine enrichments. Plasma [1-13C]leucine enrichments in the arterial and venous compartments are displayed for the whey (a), caseinate (b), and control trial (c). Data were analyzed with 2-factor (group × time) ANOVA with Student–Newman–Keuls post hoc test in case of significance. Significant interaction was observed in the arterial compartment (P < 0.01), and in the venous compartment, main effects of group (P < 0.05) and time (P < 0.001) were observed. Values represent mean ± SE
Leucine kinetics and metabolism
Leucine NB was significantly elevated after the intervention in the whey and caseinate groups with the whey intake resulting in the highest response at 30 and 60 min (P < 0.001; Fig. 3a) reaching values of 128 ± 26 and 101 ± 37 nmol min−1 100 g LBM −1leg , respectively, as compared to −2 ± 3 nmol min−1 100 g LBM −1leg at baseline (P < 0.05). Caseinate intake induced a more moderate elevation in NB, which, though, peaked together with whey at 30 min (62 ± 9 nmol min−1 100 g LBM −1leg ) and lasted until 120 min as compared to baseline and control (P < 0.05). Local leg leucine oxidation was significantly elevated above baseline at 90 and 120 min in the whey group (P < 0.05; Fig. 3b), and a tendency was observed at 180–300 min in the caseinate group (P < 0.10). Leucine oxidation was most pronounced after the whey intake at 90 and 120 min as compared to the caseinate intake (P < 0.05). Leg blood CO2 content and enrichment are displayed in Fig. 3c and d.
Leg leucine net balance and oxidation. Leucine net balance (NB) a and oxidation b at baseline (−60 min) and in the recovery period from 30 to 360 min are displayed. Data were analyzed with 2-factor (group × time) ANOVA with Student–Newman–Keuls post hoc test in case of significance. For leucine NB and oxidation, significant interactions were observed (P < 0.001), and solid (whey) and dashed (caseinate) lines indicate time points being significantly higher than baseline (P < 0.05). *Whey group significantly different from control, ¤whey group significantly different from caseinate, and #caseinate group significantly different from control, all P < 0.05. Leg blood CO2 contents c and leg blood 13CO2 enrichments d are illustrated as the underlying basis of the leg leucine oxidation calculations. Values represent mean ± SE
Total leg leucine oxidation in the recovery period represents 44 and 46 % of leucine NB in the whey and caseinate groups, respectively. In the control group, the leucine oxidation was markedly higher (254 %) than NB indicating oxidation from proteolysis derived leucine. AUC measurements on leucine NB, oxidation, and net NOLD were significantly higher in the protein groups as compared to the control group (P < 0.05−0.001; Fig. 4). No differences were observed between whey and caseinate.
Total recovery period responses of leg leucine metabolism. Area under the curves for leucine net balance (LEU NB), oxidation (LEU OXI), and net non-oxidative leucine disposal (LEU NOLD) are displayed. Group data were analyzed with 1-factor non-repeated measure ANOVA with Student–Newman–Keuls post hoc test in case of significance. Main group effects were for leucine NB: P < 0.001, leucine oxidation: P < 0.01, and net NOLD: P < 0.01. *, **, and *** indicate differences compared to control of P < 0.05, P < 0.01, and P < 0.001, respectively. Values represent mean ± SE
An interaction was observed for leucine R d (P < 0.05; Fig. 5a). R d was significantly higher in the whey group as compared to caseinate and control at 30 min and as compared to control at 60 min (both P < 0.05). At 30–60 min, the whey group displayed a higher R d as compared to baseline (P < 0.05). Leucine R a showed only main effect of time in the recovery period (P < 0.01; Fig. 5b).
Leg leucine kinetics. Leucine rate of disappearance (R d) (a) and rate of appearance (R a) (b) are displayed. Data were analyzed with 2-factor (group × time) ANOVA with Student–Newman–Keuls post hoc test in case of significance. For leucine Rd, significant interaction was observed (P < 0.05), and in the whey group, the solid line indicates time points being significantly higher than baseline (P < 0.05). *Whey group significantly different from control and ¤whey group significantly different from caseinate, both P < 0.05. Leucine Ra only showed a main effect of time (P < 0.01), where #indicate time points different from baseline (P < 0.05). Values represent mean ± SE
IGF-I, FOXO, and atrogene mRNA expressions
For IGF-IEa and IGF-IEb, general time effects were observed (P < 0.05; Fig. 6a, b). For IGF-IEa, the 210-min time point were significantly lower than 60 and 360 min (P < 0.05), and for IGF-IEb, the 360-min time point was significantly higher than −60 and 60 min (P < 0.05). For IGF-IEa, main effect of groups was observed revealing that the mRNA expression of the whey group was significantly higher than caseinate and control (P < 0.05). The mRNA expression of IGF-IEc remained unchanged (Fig. 6c).
IGF-I mRNA expressions. Local muscle IGF-I mRNA expressions are shown for isoform Ea (a), Eb (b), and Ec (c). Values are presented for the post-exercise biopsy time points 60, 210, and 360 min relative to baseline values at −60 min as geo means ± back-transformed SE. Log-transformed data were analyzed with 2-factor ANOVA (group × time), and main effects are displayed in the figure. For IGF-IEa, the post hoc test showed that whey > caseinate and whey > control (Student–Newman–Keuls test, P < 0.05). Time point effects were as follows: *significant different from 60 and 360 min and #significant different from −60 and 60 min (Student–Newman–Keuls test, P < 0.05)
The mRNA expression of FOXO1A and MuRF1 was upregulated at 60 and 210 min as compared to −60 min (P < 0.05; Fig. 7a, c). In contrast, mRNA expressions of FOXO3 and Atrogin1 were reduced at 210 and 360 min as compared to −60 min (P < 0.05; Fig. 7b, d). In addition, mRNA expression of FOXO3 was upregulated at 60 min (P < 0.05). The mRNA expression of FOXO4 remained unchanged (Fig. 7e). No significant differences were observed between groups. RPLP0 is illustrated in Fig. 7f.
FOXO and atrogene mRNA expressions. Local muscle mRNA expressions of FOXO1A (a), FOXO3 (b), muscle RING finger 1 (MuRF1) (c), Atrogin1 (d), FOXO4 (e), and RPLP0 (f) are presented for the post-exercise biopsy time points 60, 210, and 360 min relative to baseline values at −60 min as geo means ± back-transformed SE. Log-transformed data were analyzed with 2-factor ANOVA (group × time), and main effects are displayed in the figure. Time point effects were as follows: *significant different from −60 min, #significant different from all other time points, ¤significant different from −60 to 360 min, and §significant different from −60 to 210 min (Student–Newman–Keuls test, P < 0.05)
Discussion
This study investigated leg leucine kinetics, NB, and oxidation following a single bout of heavy resistance exercise and ingestion of whey, caseinate, or a non-caloric control drink. The main finding was that whey protein supplementation resulted in the most pronounced effect early in the recovery. However, the effect was equal to the response following caseinate supplementation when comparing the averaged responses over the entire 6-h recovery period. Both proteins resulted in a significantly increased anabolic response as compared to the non-caloric control drink.
Leg leucine net balance
Protein ingestion resulted in positive leg leucine NB with temporal differences between the milk protein types. There were marked differences between whey, caseinate, and control at 30 and 60 min. Nevertheless, calculated as the total response in the recovery period, we observed no differences between the protein types, which, however, were significantly higher than control. Although comparable data exist [19, 43], we here report results including local leg leucine oxidation. It could be hypothesized that the different leucine contents in the proteins simply could be the main reason for the different leucine NB between the groups observed in the early part of the recovery period. The leucine content in 100 g of protein is 11.8 g in whey and 8.8 g in caseinate. At 60 min, the arterial and venous plasma leucine concentrations were higher in the whey group relative to the caseinate. However, as the total leucine AUC responses in the total recovery period were identical between whey and caseinate [17], the major determinants of the different temporal leucine kinetics and metabolism are the relative digestion and absorption properties. Furthermore, a high digestion and absorption rate and high leucine content might not be the only determinants for a positive net protein synthesis as it very recently has been shown that sodium caseinate is superior to whey on a whole-body level in relation cycle exercise in elderly [44].
Leg leucine metabolism
Leg leucine oxidation peaked 120 min after exercise in the whey supplementation group and after 300 min in the caseinate group. No change was observed in the control group. AUC for leucine oxidation after exercise and protein intake showed similar total oxidation between whey and caseinate, which was significantly higher than control. Our results on leg muscle leucine oxidation show that a considerable amount (~45 %) of the leucine taken up by the leg after protein ingestion is directed to oxidation and therefore not incorporated into structural proteins. In particular, the marked leucine uptake early in the recovery period following exercise and whey intake increased leucine oxidation. On the other hand, ingestion of the slow digested caseinate resulted in a delayed and more moderate leucine oxidation. However, a summation of the total 30–360 min post-exercise leucine oxidation showed no difference between the two milk proteins. Therefore, a rather large part of leucine taken up by the leg is directed to energy metabolism and not utilized for protein synthesis, and the temporal diversity in oxidation rates may very well reflect the intracellular leucine availability [19]. These leg muscle specific leucine oxidation data certainly agree with whole-body measurements at rest following bolus intakes of whey and casein [12, 14]. Although speculatively, a certain leucine concentration level is required to maximally stimulate the protein synthesis machinery. This is supported by recent results showing that following resistance exercise a rapid aminoacidemia induced by a single bolus ingestion of whey is required to maximally stimulate MPS as compared to an equal amount of whey provided in ten small boluses [45]. Since the total MPS measured as FSR [17] and total leucine NB during the whole recovery period are equally increased after the protein drinks compared to control together with temporal differences in leucine concentration and oxidation, it can be argued that the threshold is not reached with the caseinate intake, whereas it may be reached in the early recovery period with the whey intake. This is supported by the fact that protein given in the same amounts as in the present study induce a MPS response above which no further effects of increasing the protein amount were observed [46, 47]. Nevertheless, based on these and our results, a larger amount (i.e. >20 g) of slowly digested protein (e.g. types of casein, milk, or meat) could hypothetically induce a greater MPS response, following resistance exercise when measured over a time-sufficient recovery period (i.e. at least 6 h).
Leg muscle protein turnover
In contrast to our previous finding of increased MPS measured with the direct incorporation technique [17], there were only minor and very transient alterations in Rd measured with the 2-compartment model in the whey group. Further, no group differences were observed for the R a being a measure of MPB. These results agree with findings following exercise and protein or amino acid supplementation. Several studies have documented that the positive regulation of muscle protein NB after exercise and amino acid/protein supplementation primarily are based on increased MPS with MPB being unchanged or only slightly elevated [1, 4, 9, 10, 30, 48, 49]. Measured with the direct incorporation model as well as with indirect compartmental modeling, MPS are robustly increased following resistance exercise with and without amino acid containing supplements [1, 3–6, 9, 30]. However, with respect to MPB, some studies demonstrate that MPB is increased together with MPS in the fasting condition [3, 4], whereas when fed EAA and carbohydrates following exercise MPB is unchanged or even tends to decrease [1, 30].
To summarize the leucine data, they confirm that whey and caseinate improve NB markedly compared to the fasted state condition and that the amount of net NOLD is markedly but evenly elevated by whey and caseinate compared to fasting control. Furthermore, with the addition of local leg measurements of leucine oxidation, our leucine NB and net NOLD data certainly do confirm previous data showing that in the total recovery period after heavy resistance exercise, there are no net anabolic differences between the whey and casein/caseinate supplementations [15, 17, 19]. However, it must not be neglected that other studies find a superior effect of whey hydrolysate compared to micellar casein in young [18] and whey to micellar casein in elderly subjects [16, 20]. These different results may, from our point of view, be ascribed primarily to differences in the respective study designs (i.e. tracer methodology and length of recovery period), while only minor to different digestive and absorptive properties between micellar casein and caseinate [50, 51]. Actually, when whey and micellar casein are ingested together (very elegantly measured with different intrinsic stable isotope labels on whey and casein), it has been shown that the protein-derived AA retention in the leg are similar from the whey and casein in the early part (80–120 min), but better from casein in later part (220–260 min) from consumption [52]. Together with our present data, this pattern of protein utilization suggests that the anabolic effects of slow digested casein/caseinate can be underestimated if the measurement period is too short [53].
IGF-I, FOXO, and atrogene mRNA expressions
We observed that IGF-IEa mRNA expression was higher in the recovery period after whey intake than after caseinate and control. This milk protein-related difference was not manifested in the mRNA expressions within the FOXO family members and the ubiquitin ligases MuRF1 and Atrogin1. In the recovery period, FOXO1A and MuRF1 were upregulated at 60 and 210 min, and, in contrast, FOXO3 and Atrogin1 were downregulated at 210 and 360 min. Furthermore, from an mRNA expression point of view, we found that FOXO1A seemed to be closely related to MuRF1 and that FOXO3 seemed to be responding similar to Atrogin1.
As both MuRF1 and Atrogin1 are known regulatory ligases in the muscle ubiquitin–proteasome system [25, 27], the present results indicate different regulatory mechanisms related to MuRF1 and Atrogin1. Likewise, other data show that MuRF1 is upregulated 1 h post-resistance exercise with Atrogin1 and muscle protein breakdown unaltered [30]. Furthermore, heavy resistance exercise induced increased MuRF1 and unchanged or decreased Atrogin1 measured 2 h post-exercise performed twice and separated by 48 h [32]. A very well-described time course pattern of MuRF1 and Atrogin1 following resistance exercise also demonstrate that MuRF1 is elevated 1, 2, and 4 h post-exercise, whereas Atrogin1 remain unaltered until a downregulation is observed at 8 and 12 h post-exercise [31]. In a recent study, the combination of resistance exercise and intake of branched-chain amino acids (BCAA) showed both common and divergent regulation of MuRF1 and Atrogin1, which in the exercised muscle, both increased in the placebo trial, whereas Atrogin1 decreased and MuRF1 increased in the BCAA supplemented trial [54]. Thus, others’ and our data clearly advocate for a differential regulation of FOXO family members, MuRF1 and Atrogin1. Some results have lead to the suggestion that MuRF1 act as a “metabolic regulatory master gene”, which are involved in the regulation of ATP synthesis and redirect glycogen synthesis to the liver under metabolic stressed conditions [55, 56]. This could explain the different pattern of expression between MuRF1 and Atrogin1 as the recovery from heavy resistance exercise induce metabolic stress not at least in the present setup where all subjects were fasted and only supplied with the protein drink. As we did not observe any significant differences between the intervention groups on muscle protein breakdown, it seems likely that the different responses of mRNA expressions of MuRF1 and Atrogin1 are part of a multifaceted regulatory system controlling the activity of the ubiquitin–proteasome system. Obviously, one suggestion could be that the upregulated MuRF1 response seems to be counteracted by the downregulation of Atrogin1, and a complementary suggestion could be that MuRF1 and Atrogin1 target different types of muscle proteins [57–60]. This interaction between atrogenes and the target protein structures could be more or less affected by the heavy resistance exercise bout and the nutrient interventions.
Method limitations
The arteriovenous 2-compartment tracer model, which is applied in the present study, does have certain limitations that should be kept in mind. Firstly, the indirect nature of the model only provides measures of amino acid kinetics (in this case leucine kinetics) between the arterial inflow and the venous outflow. It is assumed that leucine disappearance solely is due to leucine utilization for protein synthesis or oxidation. However, changes in free leucine pool sizes in the leg, which is indeed relevant in feeding studies, will under- or over-estimate rates of MPS (R d) and MPB (R a). To correct for pool size changes, frequent muscle tissue sampling is necessary, which though in most human settings are impossible. R d and R a can also be wrongly estimated due to intracellular reutilization, namely when amino acids enter the free pool from protein breakdown and directly are reincorporated into muscle proteins without entering the free pool in plasma. However, the AA NB is not affected by the amount of intracellular recycling. Furthermore, it is assumed that the chosen AA tracer is representative of all amino acids and muscle accounts for the total leg metabolism. Secondly, but importantly, no specific information regarding specific muscle proteins are provided by the arteriovenous 2-compartment model across the leg. To get direct measures of specific proteins (e.g. the structural myofibrillar proteins), other tracer-based models can be used [17, 39, 61, 62]. Specific muscle protein synthesis rates can be measured by the direct incorporation model, which gives a fractional synthesis rate in %/h [17, 39], and, recently, we have validated a model allowing a measure of the fractional breakdown rate of specific muscle proteins [61, 62]. Despite this discussion, the 2-compartment model is generally accepted to synchronously describe MPS and MPB with a high time resolution.
Conclusions
Total protein NB in the 6-h recovery period was equally affected after whey and caseinate intake with underlying temporal differences showing a high transient response after whey and a more moderate response following caseinate intake. The mRNA expression data for FOXO, MuRF1, and Atrogin1 showed no differences between the protein groups and showed different regulatory responses in the ubiquitin ligases MuRF1 and Atrogin1, which, respectively, were up- and downregulated in the recovery period. Overall, whey and caseinate are equally effective in eliciting an anabolic response following, a single bout of heavy resistance exercise together with marked temporal differences.
References
Biolo G, Tipton KD, Klein S, Wolfe RR (1997) An abundant supply of amino acids enhances the metabolic effect of exercise on muscle protein. Am J Physiol Endocrinol Metab 273:E122–E129
Kumar V, Atherton P, Smith K, Rennie MJ (2009) Human muscle protein synthesis and breakdown during and after exercise. J Appl Physiol 106:2026–2039
Phillips SM, Tipton KD, Aarsland A, Wolf SE, Wolfe RR (1997) Mixed muscle protein synthesis and breakdown after resistance exercise in humans. Am J Physiol Endocrinol Metab 273:E99–E107
Biolo G, Maggi SP, Williams BD, Tipton KD, Wolfe RR (1995) Increased rates of muscle protein turnover and amino acid transport after resistance exercise in humans. Am J Physiol Endocrinol Metab 268:E514–E520
Burd NA, Holwerda AM, Selby KC, West DW, Staples AW, Cain NE, Cashaback JG, Potvin JR, Baker SK, Phillips SM (2010) Resistance exercise volume affects myofibrillar protein synthesis and anabolic signalling molecule phosphorylation in young men. J Physiol 588:3119–3130
Chesley A, MacDougall JD, Tarnopolsky MA, Atkinson SA, Smith K (1992) Changes in human muscle protein synthesis after resistance exercise. J Appl Physiol 73:1383–1388
Aagaard P, Andersen JL, Dyhre-Poulsen P, Leffers AM, Wagner A, Magnusson SP, Halkjaer-Kristensen J, Simonsen EB (2001) A mechanism for increased contractile strength of human pennate muscle in response to strength training: changes in muscle architecture. J Physiol 534:613–623
Holm L, Reitelseder S, Pedersen TG, Doessing S, Petersen SG, Flyvbjerg A, Andersen JL, Aagaard P, Kjaer M (2008) Changes in muscle size and MHC composition in response to resistance exercise with heavy and light loading intensity. J Appl Physiol 105:1454–1461
Borsheim E, Tipton KD, Wolf SE, Wolfe RR (2002) Essential amino acids and muscle protein recovery from resistance exercise. Am J Physiol Endocrinol Metab 283:E648–E657
Tipton KD, Ferrando AA, Phillips SM, Doyle D Jr, Wolfe RR (1999) Postexercise net protein synthesis in human muscle from orally administered amino acids. Am J Physiol Endocrinol Metab 276:E628–E634
World Health Organization (2007) Protein and amino acid requirements in human nutrition: report of a joint WHO/FAO/UNU expert consultation. WHO technical report series #935, Geneva
Boirie Y, Dangin M, Gachon P, Vasson MP, Maubois JL, Beaufrere B (1997) Slow and fast dietary proteins differently modulate postprandial protein accretion. Proc Natl Acad Sci USA 94:14930–14935
Calbet JA, Holst JJ (2004) Gastric emptying, gastric secretion and enterogastrone response after administration of milk proteins or their peptide hydrolysates in humans. Eur J Nutr 43:127–139
Dangin M, Boirie Y, Garcia-Rodenas C, Gachon P, Fauquant J, Callier P, Ballevre O, Beaufrere B (2001) The digestion rate of protein is an independent regulating factor of postprandial protein retention. Am J Physiol Endocrinol Metab 280:E340–E348
Dideriksen KJ, Reitelseder S, Petersen SG, Hjort M, Helmark IC, Kjaer M, Holm L (2011) Stimulation of muscle protein synthesis by whey and caseinate ingestion after resistance exercise in elderly individuals. Scand J Med Sci Sports 21:e372–e383
Pennings B, Boirie Y, Senden JM, Gijsen AP, Kuipers H, van Loon LJ (2011) Whey protein stimulates postprandial muscle protein accretion more effectively than do casein and casein hydrolysate in older men. Am J Clin Nutr 93:997–1005
Reitelseder S, Agergaard J, Doessing S, Helmark IC, Lund P, Kristensen NB, Frystyk J, Flyvbjerg A, Schjerling P, van Hall G, Kjaer M, Holm L (2011) Whey and casein labeled with L-[1-13C]leucine and muscle protein synthesis: effect of resistance exercise and protein ingestion. Am J Physiol Endocrinol Metab 300:E231–E242
Tang JE, Moore DR, Kujbida GW, Tarnopolsky MA, Phillips SM (2009) Ingestion of whey hydrolysate, casein, or soy protein isolate: effects on mixed muscle protein synthesis at rest and following resistance exercise in young men. J Appl Physiol 107:987–992
Tipton KD, Elliott TA, Cree MG, Wolf SE, Sanford AP, Wolfe RR (2004) Ingestion of casein and whey proteins result in muscle anabolism after resistance exercise. Med Sci Sports Exerc 36:2073–2081
Burd NA, Yang Y, Moore DR, Tang JE, Tarnopolsky MA, Phillips SM (2012) Greater stimulation of myofibrillar protein synthesis with ingestion of whey protein isolate v. micellar casein at rest and after resistance exercise in elderly men. Br J Nutr 108:958–962
Glass DJ (2005) Skeletal muscle hypertrophy and atrophy signaling pathways. Int J Biochem Cell Biol 37:1974–1984
Sandri M (2008) Signaling in muscle atrophy and hypertrophy. Physiology (Bethesda) 23:160–170
Sandri M, Sandri C, Gilbert A, Skurk C, Calabria E, Picard A, Walsh K, Schiaffino S, Lecker SH, Goldberg AL (2004) Foxo transcription factors induce the atrophy-related ubiquitin ligase atrogin-1 and cause skeletal muscle atrophy. Cell 117:399–412
Stitt TN, Drujan D, Clarke BA, Panaro F, Timofeyva Y, Kline WO, Gonzalez M, Yancopoulos GD, Glass DJ (2004) The IGF-1/PI3 K/Akt pathway prevents expression of muscle atrophy-induced ubiquitin ligases by inhibiting FOXO transcription factors. Mol Cell 14:395–403
Bodine SC, Latres E, Baumhueter S, Lai VK, Nunez L, Clarke BA, Poueymirou WT, Panaro FJ, Na E, Dharmarajan K, Pan ZQ, Valenzuela DM, DeChiara TM, Stitt TN, Yancopoulos GD, Glass DJ (2001) Identification of ubiquitin ligases required for skeletal muscle atrophy. Science 294:1704–1708
Dehoux MJ, van Beneden RP, Fernandez-Celemin L, Lause PL, Thissen JP (2003) Induction of MafBx and Murf ubiquitin ligase mRNAs in rat skeletal muscle after LPS injection. FEBS Lett 544:214–217
Gomes MD, Lecker SH, Jagoe RT, Navon A, Goldberg AL (2001) Atrogin-1, a muscle-specific F-box protein highly expressed during muscle atrophy. Proc Natl Acad Sci USA 98:14440–14445
Nikawa T, Ishidoh K, Hirasaka K, Ishihara I, Ikemoto M, Kano M, Kominami E, Nonaka I, Ogawa T, Adams GR, Baldwin KM, Yasui N, Kishi K, Takeda S (2004) Skeletal muscle gene expression in space-flown rats. FASEB J 18:522–524
Wray CJ, Mammen JM, Hershko DD, Hasselgren PO (2003) Sepsis upregulates the gene expression of multiple ubiquitin ligases in skeletal muscle. Int J Biochem Cell Biol 35:698–705
Glynn EL, Fry CS, Drummond MJ, Dreyer HC, Dhanani S, Volpi E, Rasmussen BB (2010) Muscle protein breakdown has a minor role in the protein anabolic response to essential amino acid and carbohydrate intake following resistance exercise. Am J Physiol Regul Integr Comp Physiol 299:R533–R540
Louis E, Raue U, Yang Y, Jemiolo B, Trappe S (2007) Time course of proteolytic, cytokine, and myostatin gene expression after acute exercise in human skeletal muscle. J Appl Physiol 103:1744–1751
Mascher H, Tannerstedt J, Brink-Elfegoun T, Ekblom B, Gustafsson T, Blomstrand E (2008) Repeated resistance exercise training induces different changes in mRNA expression of MAFbx and MuRF-1 in human skeletal muscle. Am J Physiol Endocrinol Metab 294:E43–E51
Churchley EG, Coffey VG, Pedersen DJ, Shield A, Carey KA, Cameron-Smith D, Hawley JA (2007) Influence of preexercise muscle glycogen content on transcriptional activity of metabolic and myogenic genes in well-trained humans. J Appl Physiol 102:1604–1611
Radegran G (1997) Ultrasound Doppler estimates of femoral artery blood flow during dynamic knee extensor exercise in humans. J Appl Physiol 83:1383–1388
Holm L, van Hall G, Rose AJ, Miller BF, Doessing S, Richter EA, Kjaer M (2010) Contraction intensity and feeding affect collagen and myofibrillar protein synthesis rates differently in human skeletal muscle. Am J Physiol Endocrinol Metab 298:E257–E269
Biolo G, Chinkes D, Zhang XJ, Wolfe RR (1992) Harry M. Vars research award. A new model to determine in vivo the relationship between amino acid transmembrane transport and protein kinetics in muscle. J Parenter Enteral Nutr 16:305–315
Biolo G, Fleming RY, Maggi SP, Wolfe RR (1995) Transmembrane transport and intracellular kinetics of amino acids in human skeletal muscle. Am J Physiol Endocrinol Metab 268:E75–E84
Fujita S, Rasmussen BB, Bell JA, Cadenas JG, Volpi E (2007) Basal muscle intracellular amino acid kinetics in women and men. Am J Physiol Endocrinol Metab 292:E77–E83
Wolfe RR, Chinkes DL (2005) Isotope tracers in metabolic research: principles and practice of kinetic analysis. Wiley, Hoboken
Matthews DE, Motil KJ, Rohrbaugh DK, Burke JF, Young VR, Bier DM (1980) Measurement of leucine metabolism in man from a primed, continuous infusion of L-[1-13C]leucine. Am J Physiol Endocrinol Metab 238:E473–E479
Tarnopolsky MA, Atkinson SA, MacDougall JD, Senor BB, Lemon PW, Schwarcz H (1991) Whole body leucine metabolism during and after resistance exercise in fed humans. Med Sci Sports Exerc 23:326–333
van Hall G (1999) Correction factors for 13C-labelled substrate oxidation at whole-body and muscle level. Proc Nutr Soc 58:979–986
Tipton KD, Elliott TA, Cree MG, Aarsland AA, Sanford AP, Wolfe RR (2007) Stimulation of net muscle protein synthesis by whey protein ingestion before and after exercise. Am J Physiol Endocrinol Metab 292:E71–E76
Engelen MPKJ, Rutten EPA, De Castro CLN, Wouters EFM, Schols AMWJ, Deutz NEP (2012) Casein protein results in higher prandial and exercise induced whole body protein anabolism than whey protein in chronic obstructive pulmonary disease. Metabolism 61:1289–1300
West DW, Burd NA, Coffey VG, Baker SK, Burke LM, Hawley JA, Moore DR, Stellingwerff T, Phillips SM (2011) Rapid aminoacidemia enhances myofibrillar protein synthesis and anabolic intramuscular signaling responses after resistance exercise. Am J Clin Nutr 94:795–803
Cuthbertson D, Smith K, Babraj J, Leese G, Waddell T, Atherton P, Wackerhage H, Taylor PM, Rennie MJ (2005) Anabolic signaling deficits underlie amino acid resistance of wasting, aging muscle. FASEB J 19:422–424
Moore DR, Robinson MJ, Fry JL, Tang JE, Glover EI, Wilkinson SB, Prior T, Tarnopolsky MA, Phillips SM (2009) Ingested protein dose response of muscle and albumin protein synthesis after resistance exercise in young men. Am J Clin Nutr 89:161–168
Rasmussen BB, Tipton KD, Miller SL, Wolf SE, Wolfe RR (2000) An oral essential amino acid-carbohydrate supplement enhances muscle protein anabolism after resistance exercise. J Appl Physiol 88:386–392
Tipton KD, Borsheim E, Wolf SE, Sanford AP, Wolfe RR (2003) Acute response of net muscle protein balance reflects 24-h balance after exercise and amino acid ingestion. Am J Physiol Endocrinol Metab 284:E76–E89
Phillips SM (2011) A comparison of whey to caseinate. Am J Physiol Endocrinol Metab 300:E610
Reitelseder S, Kjaer M, Holm L (2011) Reply to letter to the editor. Am J Physiol Endocrinol Metab 300:E611–E612
Soop M, Nehra V, Henderson GC, Boirie Y, Ford GC, Nair KS (2012) Coingestion of whey protein and casein in a mixed meal: demonstration of a more sustained anabolic effect of casein. Am J Physiol Endocrinol Metab 303:E152–E162
Jonker R, Engelen MPKJ, Deutz NEP (2012) Role of specific dietary amino acids in clinical conditions. Br J Nutr 108:S139–S148
Borgenvik M, Apro W, Blomstrand E (2012) Intake of branched-chain amino acids influences the levels of MAFbx mRNA and MuRF-1 total protein in resting and exercising human muscle. Am J Physiol Endocrinol Metab 302:E510–E521
Hirner S, Krohne C, Schuster A, Hoffmann S, Witt S, Erber R, Sticht C, Gasch A, Labeit S, Labeit D (2008) MuRF1-dependent regulation of systemic carbohydrate metabolism as revealed from transgenic mouse studies. J Mol Biol 379:666–677
Koyama S, Hata S, Witt CC, Ono Y, Lerche S, Ojima K, Chiba T, Doi N, Kitamura F, Tanaka K, Abe K, Witt SH, Rybin V, Gasch A, Franz T, Labeit S, Sorimachi H (2008) Muscle RING-finger protein-1 (MuRF1) as a connector of muscle energy metabolism and protein synthesis. J Mol Biol 376:1224–1236
Lagirand-Cantaloube J, Offner N, Csibi A, Leibovitch MP, Batonnet-Pichon S, Tintignac LA, Segura CT, Leibovitch SA (2008) The initiation factor eIF3-f is a major target for atrogin1/MAFbx function in skeletal muscle atrophy. EMBO J 27:1266–1276
Foletta VC, White LJ, Larsen AE, Leger B, Russell AP (2011) The role and regulation of MAFbx/atrogin-1 and MuRF1 in skeletal muscle atrophy. Pflugers Arch 461:325–335
Lagirand-Cantaloube J, Cornille K, Csibi A, Batonnet-Pichon S, Leibovitch MP, Leibovitch SA (2009) Inhibition of atrogin-1/MAFbx mediated MyoD proteolysis prevents skeletal muscle atrophy in vivo. PLoS ONE 4:e4973
Cohen S, Brault JJ, Gygi SP, Glass DJ, Valenzuela DM, Gartner C, Latres E, Goldberg AL (2009) During muscle atrophy, thick, but not thin, filament components are degraded by MuRF1-dependent ubiquitylation. J Cell Biol 185:1083–1095
Holm L, Kjaer M (2010) Measuring protein breakdown in individual proteins in vivo. Curr Opin Clin Nutr Metab Care 13:526–531
Holm L, O’Rourke B, Ebenstein D, Toth MJ, Bechshoeft R, Holstein-Rathlou N-H, Kjaer M, Matthews DE (2013) Determination of steady state protein breakdown rate in vivo by the disappearance of protein-bound tracer-labeled amino acids: a method applicable in humans. Am J Physiol Endocrinol Metab. doi:10.1152/ajpendo.00579.2012
Acknowledgments
We thank our voluntary participants for their time and effort and Ann-Marie Sedstrøm, Camilla Hansen, and Ann-Christina Reimann for technical assistance during sampling and analyses. Additionally, Flemming Jessen and Nina Pluszek at Clinical Metabolomics Core Facility, Rigshospitalet, Copenhagen, are specially thanked for great assistance with the mass spectrometry. The Danish Dairy Research Foundation, H:S Research Foundation (Copenhagen University Hospitals), Danish Medical Research Council, and The Ministry of Culture Committee on Sports Research supported this work financially.
Conflict of interest
The authors declare no conflicts of interest, financial or otherwise.
Author information
Authors and Affiliations
Corresponding author
Electronic supplementary material
Below is the link to the electronic supplementary material.
Rights and permissions
About this article
Cite this article
Reitelseder, S., Agergaard, J., Doessing, S. et al. Positive muscle protein net balance and differential regulation of atrogene expression after resistance exercise and milk protein supplementation. Eur J Nutr 53, 321–333 (2014). https://doi.org/10.1007/s00394-013-0530-x
Received:
Accepted:
Published:
Issue Date:
DOI: https://doi.org/10.1007/s00394-013-0530-x