Abstract
We tested the hypothesis that development of the Antarctic urchin Sterechinus neumayeri under future ocean conditions of warming and acidification would incur physiological costs, reducing the tolerance of a secondary stressor. The aim of this study is twofold: (1) quantify current austral spring temperature and pH near sea urchin habitat at Cape Evans in McMurdo Sound, Antarctica and (2) spawn S. neumayeri in the laboratory and raise early developmental stages (EDSs) under ambient (−0.7 °C; 400 µatm pCO2) and future (+2.6 °C; 650 and 1,000 µatm pCO2) ocean conditions and expose four EDSs (blastula, gastrula, prism, and 4-arm echinopluteus) to a one hour acute heat stress and assess survivorship. Results of field data from 2011 to 2012 show extremely stable inter-annual pH conditions ranging from 7.99 to 8.08, suggesting that future ocean acidification will drastically alter the pH-seascape for S. neumayeri. In the laboratory, S. neumayeri EDSs appear to be tolerant of temperatures and pCO2 levels above their current habitat conditions. EDSs survived acute heat exposures >20 °C above habitat temperatures of −1.9 °C. No pCO2 effect was observed for EDSs reared at −0.7 °C. When reared at +2.6 °C, small but significant pCO2 effects were observed at the blastula and prism stage, suggesting that multiple stressors are more detrimental than single stressors. While surprisingly tolerant overall, blastulae were the most sensitive stage to ocean warming and acidification. We conclude that S. neumayeri may be unexpectedly physiologically tolerant of future ocean conditions.
Similar content being viewed by others
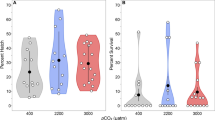
Explore related subjects
Discover the latest articles, news and stories from top researchers in related subjects.Avoid common mistakes on your manuscript.
Introduction
Due to properties of persistent cold seawater, high-latitude marine ecosystems are ranked to be among the most sensitive regions to global change (Orr et al. 2005; Peck 2005; McClintock et al. 2008; Fabry et al. 2009; Turley et al. 2010). The Southern Ocean is expected to significantly change with respect to multiple factors within decades, due to anthropogenic release of carbon dioxide (CO2) to the atmosphere (Orr et al. 2005; McNeil and Matear 2008). Specifically, unprecedented rates of ocean warming and acidification are predicted (IPCC 2007; Stammerjohn et al. 2008; Hönisch et al. 2012; Marcott et al. 2013). The fingerprints of this change are already being documented in Antarctic marine ecosystems (McClintock et al. 2008; Montes-Hugo et al. 2009; Bednaršek et al. 2012; Naveen et al. 2012; Steinberg et al. 2012). Due to the rate of environmental change and narrow adaptive capacity of Antarctic marine organisms, future shifts in the structure and function of Antarctic ecosystems are expected to be significant (Peck 2005; Fabry et al. 2009). A critical research priority is to assess the adaptive capacity of Antarctic organisms to future complex changes, which are often not considered together (Boyd 2011). In this light, the aim of this study was to assess the physiological cost of development of a circum-Antarctic marine invertebrate, the sea urchin Sterechinus neumayeri, under future multi-stressor scenarios.
Assessing the adaptive capacity (sensu Dawson et al. 2011) of Antarctic organisms is a critical research priority as the rate of change of waters surrounding Antarctica is expected to be rapid. As atmospheric CO2 dissolves into the surface layers of the ocean, due to differential partial pressures in the atmosphere and seawater, ocean pCO2 increases and pH decreases. Atmospheric pCO2 exceeded 400 ppm in May 2013 (http://www.esrl.noaa.gov/gmd/ccgg/trends/); an increase from 280 ppm pre-industrial time (Feely 2004). The Southern Ocean is the fastest acidifying ocean on the planet with an annual pCO2 increase of 2.13 ± 0.64 µatm year−1 since the 1980s (Takahashi et al. 2009). Atmospheric CO2 is predicted to reach 1,000 ppm by 2100 resulting in a decrease of 0.4 ocean pH (IPCC 2007). However, biological ramifications in the Southern Ocean may become apparent earlier with seasonal aragonite undersaturation predicted to occur when atmospheric CO2 levels reach 450 ppm, as early as 2030 (McNeil and Matear 2008). The Southern Ocean has warmed at twice the rate of global warming: 0.17 °C since the 1950s (Fyfe 2006; Gille 2002), and sea surface temperature is predicted to rise by an additional 2.6 °C in the next 90 years (IPCC 2007).
The ‘double whammy’ of ocean acidification and ocean warming will create unprecedented marine environments and is expected to severely impact functional traits and physiological processes of polar species (Fabry et al. 2009; Sewell and Hofmann 2011). In general, species and populations may exhibit three types of responses to environmental change: (1) migrate with the changing climate envelope (Parmesan and Yohe 2003), (2) genetically adapt (Hoffmann and Sgrò 2011), and (3) acclimatize using current physiological plasticity (Gienapp et al. 2008; Visser 2008; Hoffmann and Sgrò 2011).
Migration to colder water, the poleward shifts observed at lower latitudes, is not an option for polar organisms. Here, changes in bathymetric distribution are also not viable as there is no thermocline that might preserve colder water at depth. Genetic adaptation may also be limited. Due to cold adaptation and thermal stability of the Southern Ocean over evolutionary timescales, some Antarctic species have lost the genetic capacity to deal with environmental change (Pörtner et al. 2012; Somero 2012). For example, the Antarctic notothenioid fish Trematomus bernacchii has lost an inducible heat shock response as a result of evolutionary adaptation to cold temperatures, whereas temperate notothenioids have retained this trait (Place et al. 2004; Hofmann et al. 2000, 2005; Clark and Peck 2009). Such evolutionary adaptations may result in reduced capacities to withstand future multi-stressor environments (Enzor et al. 2013). Additionally, slow growth and long generation times of Antarctic invertebrate species reduce the potential for rapid genetic adaptation that may be required to flourish in a future ocean (Peck 2005; Pörtner et al. 2007). Studies on marine invertebrates in general suggest that they are already operating at the limits of their thermal tolerance (Sunday et al. 2012), and so further acclimatization to warming may be limited, especially in polar species (Peck et al. 2009). Thus, success of Antarctic marine species in a future ocean likely depends on current physiological plasticity and acclimatization capacity of functional traits. The importance of present-day tolerances and functional traits has been emphasized in other ecosystems when predicting climate change responses (Buckley and Kingsolver 2012; Chown 2012).
To investigate the breadth of current plasticity in a polar marine invertebrate with respect to seawater temperature and pH, we studied the early developmental stages (EDSs) of the Antarctic sea urchin S. neumayeri. S. neumayeri is an ideal study organism for ocean change experimental biology. With an extensive biogeographic range, it is the most abundant echinoid on the shallow Antarctic benthos and a critical member of the circum-Antarctic near-shore marine ecosystem (Bosch et al. 1987). Adults can easily be spawned in the laboratory, and larvae can readily be cultured (Bosch et al. 1987). In general, EDSs are predicted to be among the most sensitive stages to environmental change (Kurihara 2008; Dupont and Thorndyke 2009; Byrne 2011), especially with respect to synergistic stressors (Harvey et al. 2013). Due to a long pelagic larval duration of up to 115 days (Bosch et al. 1987), S. neumayeri spend a significant amount of time under direct influence of seawater conditions during its potentially most sensitive life history stage. Additionally, the slow development of S. neumayeri provides a unique opportunity to assess the physiological plasticity in response to multi-stressors at different EDSs. Previous studies of S. neumayeri EDSs have shown reduced growth (Clark et al. 2009; Byrne et al. 2013; Yu et al. 2013) and enhanced larval asymmetry (Byrne et al. 2013) under elevated pCO2 conditions, suggesting that there is in fact a physiological cost of development under these conditions.
The goal of this study was to assess, in general, the sensitivity of S. neumayeri EDSs (from present-day spawning) to future abiotic conditions expected for the Southern Ocean. In order to assess its current physiological plasticity, we asked whether early development under elevated temperature and pCO2 came at a cost of other physiological tolerances, in this case the tolerance of an acute heat stress. As an example, temperate sea urchin and abalone larvae reared under elevated pCO2 showed reduced heat stress response following a one hour (1 h) acute heat exposure (O’Donnell et al. 2008; Zippay and Hofmann 2010a). This suggests that there are trade-offs associated with development at high pCO2 where energy is diverted away from the cellular stress response, potentially leaving the organism ill-equipped to deal with secondary stressors. Use of acute heat stress tests and laboratory-determined thermal tolerance limits can provide valuable insight into species’ physiological trade-offs and energy allocations, current physiological plasticity and acclimation capacities (Tomanek 2010; Terblanche et al. 2011) and ultimately, resilience in the face of climate change (Chown and Gaston 2008; Barnes et al. 2010; Buckley and Kingsolver 2012; Chown 2012).
During austral spring when natural spawning occurs, we raised larvae in the laboratory at McMurdo Station, Antarctica, under six multi-stressor scenarios (two temperatures and three pCO2 levels) and exposed four developmental stages to a 1 h heat stress test and assessed survivorship. We hypothesized that elevated pCO2 and high temperature incur a physiological cost to S. neumayeri EDSs and predicted that embryos and larvae will have reduced survivorship following a 1 h temperature challenge compared to larvae reared under ambient conditions. In order to place the laboratory findings in an environmentally relevant context, we also measured field pH and temperature in the water column above a sea urchin population for the duration of the larval culturing. Here, we hope to address two questions: (1) what is the natural variability in the local seawater with respect to pH and (2) does development under future conditions of ocean acidification and warming alter the acute heat tolerance of S. neumayeri EDSs?
Materials and methods
Field pH measurements
In order to parameterize the CO2 manipulation experiments of larval cultures, we measured pH near a S. neumayeri population using a SeaFET sensor with a Honeywell Durafet® pH electrode (Martz et al. 2010). SeaFET pH sensors were deployed above the benthos at 18 m depth on a 27 m benthic mooring at Cape Evans, Ross Island, Antarctica, at the sea urchin collection site (S 77°38.060′, E 166°24.918′) from late October through November, both years during 24 h daylight. In 2011, a temperature sensor was also deployed (Sea Bird Electronics SBE-37 SM, Matson 2012).
Raw voltage recorded by the SeaFET was converted to pHtotal using one discrete seawater sample per sensor deployment. Single discrete calibration samples were collected via SCUBA following standard operating procedures (SOP) 1 and processed for spectrophotometric pH analysis (total hydrogen ion concentration pH scale) at 25 °C following SOP 6b (Dickson et al. 2007). Salinity was measured using a YSI 3100 Conductivity Instrument. Total alkalinity was determined according to SOP 3b, using an open-cell titrator (Mettler-Toledo T50) after measuring certified reference materials (CRMs) seawater standards from Dr. Andrew M. Dickson at Scripps Institution of Oceanography to within 10 µmol kg−1 accuracy (Dickson et al. 2007). In situ pHtotal of the calibration sample was calculated in CO2Calc (Robbins et al. 2010) using in situ temperature recorded by the SeaFET at the time of sample collection and CO2 constants from Mehrbach et al. (1973) refit by Dickson and Millero (1987).
Accuracy of SeaFET data depends on the quality of the calibration sample and is estimated to be ~0.01 pH as compared to SOP 6b processing of CRMs (data not shown, Matson et al. 2011). pH was recorded every hour (2011) or 30 min (2012) and reported as hourly data after applying a 1 h low-pass filter.
Animal collection and larval cultures
In order to raise sea urchin EDSs under multi-stressor scenarios of temperatures and pCO2 conditions, cultures were reared at −0.7 °C in 2011 and +2.6 °C in 2012 (hereinafter referred to as ‘cold’ and ‘warm’ cultures) at three pCO2 levels (400, 650, and 1,000 µatm) as described in Yu et al. (2013). The warm culture temperature was chosen to be an extreme future scenario. Adult S. neumayeri were collected from the benthos by SCUBA at Cape Evans, McMurdo Sound, Antarctica (S 77°38.060′, E 166°24.918′), in October 2011 and 2012 during the spawning season (Pearse and Giese 1966; Brey et al. 1995; Stanwell-Smith and Peck 1998). Depth of the collection site was approximately 20 m. Adults were transported in coolers to McMurdo Station and maintained in ~−1 °C flow-through seawater tables for approximately two weeks until spawning.
Adult S. neumayeri were induced to spawn by injection of ~1 ml ice-cold 0.55 M KCl. Females were inverted over a beaker containing filtered seawater (FSW) on ice, to collect eggs; sperm was collected dry directly from the gonopores. Sperm and eggs were kept on wet ice until use. After successful test fertilization assays, eggs from 20 (15) females were pooled and fertilized using diluted sperm from one male to >90 % fertilization success for the cold (warm) culture. Embryos were split between culture buckets prefilled with control and two CO2-acidified seawater treatments as described in Yu et al. (2013). Culture vessels were stocked at ~160,000 embryos/12 l.
Experimental seawater acidification
For experimental sea urchin cultures, seawater was acidified with CO2 gas. Control and CO2-acidification of 0.32 µm-FSW followed methods modified from Fangue et al. (2010) and as described in Yu et al. (2013), with the exception that seawater tubing was not insulated. Briefly, pure CO2 gas was mixed with dry air to desired levels and subsequently dissolved into FSW in three reservoir buckets by a venturi injector. We aimed for a control pCO2 level of 400 µatm and treatment pCO2 levels of 650 and 1,000 µatm in five replicate culture vessels for a total of 15 culture vessels. Reservoir and culture vessels were thermally controlled in flow-through seawater tables with ambient seawater (cold culture) and temperature-control immersion heaters (Process Technology) (warm culture).
Reservoir buckets were sampled daily for temperature, salinity, total alkalinity and pH according to methods listed under ‘field pH measurements’ for discrete seawater samples, with the exception that temperature was measured with a wire probe (Fluke 52 K/J Thermometer). Culture vessels were sampled daily for temperature and pH. In situ pHtotal and carbonate parameters were calculated in CO2Calc (Robbins et al. 2010), using batch processing and CO2 constants from Mehrbach et al. (1973) refit by Dickson and Millero (1987).
Survivorship assays
Acute thermal tolerance of S. neumayeri was tested at four stages of sea urchin early development—hatched blastula, mid-gastrula, prism, and 4-arm echinopluteus (hereinafter ‘pluteus’)—using a survivorship assay modified from Hammond and Hofmann (2010). A thermal gradient was established by cooling and warming two ends of an aluminum block containing 60 holes fabricated to fit 20 ml scintillation vials. Thermal tolerance was tested at nine temperatures, with two technical replicates per acute heat stress temperature and pCO2 group for each stage (Table 1). Unlike ecologically relevant culture temperatures of −0.7 and +2.6 °C, acute heat stress temperatures (up to 25 °C) were chosen to study the physiology of EDSs and do not represent temperatures likely to be encountered in the field. For optimum temperature range, two assays were required for each development stage. As a matter of protocol for all CO2 treatments, the cooler assay was always run before the warmer assay. All assays were conducted in the Crary Laboratories’ walk-in environmental rooms maintained at −1 and +4 °C for the cold and warm culture, respectively.
Prior to each assay, 20 ml scintillation vials were filled with 5 ml control seawater, capped, and allowed to equilibrate to temperatures within the aluminum block for two hours. Embryos and larvae were collected from all five replicate culture vessels, concentrated through 64-µm Nitex mesh and pooled. Concentrated larvae were kept on ice in environmental rooms until used for the survivorship assay at ~−1 and 0 °C for the cold and warm culture, respectively. Approximately 220–300 larvae were transferred to each temperature-equilibrated vial in no more than 250 μl. Location was randomized by CO2 treatment within the aluminum block, and time of embryos/larvae addition was noted. Temperatures in the vials were measured immediately prior to embryos/larvae addition and upon removal of vials from the aluminum block after 1 h. The mean of these two temperatures is reported (Table 1). The brief temperature change induced by the addition of embryos/larvae was negligible. Embryos/larvae were allotted a 20 h recovery period at control temperatures (on ice in a −1 °C environmental room for the cold culture, and +2 °C water bath for the warm culture). For the first blastula stage assay of the cold culture, addition of larvae to the vials was staggered by 30 min for each temperature (cold to warm) and scored in order. For all remaining assays, larvae were added consecutively by temperature and scored randomly.
After a 20 h recovery period, larvae were concentrated by reverse filtration using a transfer pipette with 64-µm Nitex mesh. The first 100 embryos/larvae viewed under a microscope on a Sedgewick Rafter counting cell slide were scored for survival in the environmental room. Abnormal embryos/larvae (due to abnormal development or evidence of cellular egression or regression) that still exhibited ciliary activity were considered dead. Tipping-point temperatures (TTs) were determined in order to compare thermal tolerance across the cold and warm cultures and are defined as the highest temperature at which both replicates of EDSs reared under control pCO2 levels have greater than 80 percent survivorship.
Statistical analysis
Survivorship data of the 1 h temperature exposure were analyzed by developmental stage and separately for cold and warm larval cultures, using a second-order logistic model with a beta-binomial distribution (JMP 9) where pCO2, temperature (T), and T 2 were considered main effects. There were no interactions between pCO2 and temperature effects at any stage, and the interaction was removed from the final model.
Results
Field pH measurements
In general, pH was remarkably stable over the period of the SeaFET deployment in austral spring. Continuous pH data were recorded during two separate field seasons: 33 days in 2011 (October 29, 2011 to November 30, 2011, see Matson 2012) and 14 days in 2012 (October 31, 2012 to November 13, 2012, this study). pH was consistent over both field seasons (Fig. 1; Table 2). During these recording intervals, pH varied from 7.99–8.08 (2011) to 8.01–8.08 (2012) with median values of 8.01 during both seasons. Mean pH in 2011 and 2012 was 8.01 ± 0.02 and 8.02 ± 0.01, respectively. Temperature in 2011 ranged from −1.90 to −1.78 °C with a mean of −1.88 ± 0.03 °C (Matson 2012). Due to the SeaFET location under fast sea ice and stable SeaFET temperature data (not shown) and previously documented temperatures (Hunt et al. 2003), we assumed a constant temperature of −1.9 °C to calculate pCO2 and carbonate ion saturation states (Ω) for the 2012 deployment (Table 2).
Conditions of the laboratory sea urchin cultures
During the laboratory portion of the experiment, pH (as controlled by CO2-mixing) and temperature in the sea urchin cultures were stable. The three CO2 treatments resulted in similar pH conditions in both the cold (2011) and warm (2012) culture (Fig. 1). Average cold and warm culture temperatures were −0.7 ± 0.3 and +2.6 ± 0.1 °C, respectively, with little variation between culture vessels. pH and pCO2 levels of the cold culture remained stable throughout the experiment (Table 3). Control pH of the cold culture was 8.038 ± 0.015 (402 ± 16 µatm pCO2) and slightly higher than observed field pH in 2011 and 2012, whereas control pH of the warm culture matched field pH at 8.009 ± 0.027 (437 ± 16 µatm pCO2). Failure of mass flow control valves on day 12 of the warm culture resulted in a one day increase of pCO2 to 2,651 ± 353 µatm in control culture vessels and decrease to 216 ± 18 µatm in the high CO2 treatment. Subsequently on day 14, medium CO2 treatment levels declined to 216 ± 18 µatm pCO2 and failed to recover for the remainder of the experiment. The warm culture pluteus stage was the only larval stage affected by the valve failures in this study. This stage was still included in the heat stress experiment.
Comparison of field pHtotal and laboratory pHtotal conditions of early developmental sea urchin cultures in 2011 (a) and 2012 (b). Field pH (black line) was measured by a SeaFET sensor at 18 m depth and above a Sterechinus neumayeri population at Cape Evans, McMurdo Sound, Antarctica. pH of CO2-acidified experimental cultures (control pCO2 = light gray line with dot symbols; medium pCO2 = dark gray circles; high pCO2 = white triangles) was measured via spectrophotometric analysis and reported as daily averages (error bars are SD). Culture temperature in 2011 was −0.7 and +2.6 °C in 2012. Culture pH in (b) is reported up to the prism stage (see text for details). Time is in Coordinated Universal Time
Developmental progression of sea urchins in experimental cultures
Overall, development of S. neumayeri larvae occurred synchronously across all CO2 treatments with development progressing faster in the culture maintained at +2.6 °C as compared to the culture maintained at −0.7 °C (Fig. 2). As measured by prevalence of >90 %, at −0.7 °C, embryos reached hatched blastula at 104 h, gastrula by day 11, prism by day 15, and pluteus by day 21. At +2.6 °C, S. neuamayeri reached these same stages at 84 h, and by day 6, 11, and 16, respectively. Sampling of developmental stages of the warm culture was timed to best match developmental stages of the cold culture in the previous year as determined by daily observation. Due to the rapid development of embryos in the warm culture, there may have been a mismatch at the gastrula stage where slightly developmentally younger stage gastrulae, with smaller archenterons, were sampled in the warm culture compared to the cold culture. Some mortality was observed across all treatments at the prism and pluteus stages during the experiment but was not quantified as the requirement for experiments, other than the one reported here, dictated the need for large numbers of larvae.
Sampling schedule for survivorship assays of Sterechinus neumayeri early developmental stages (blastula, gastrula, prism, and 4-arm pluteus) reared at −0.7 °C (white dots) and +2.6 °C (black dots). Sampling was conducted once >90 % of embryos or larvae reached the stage of interest. Split dot represents sampling of both cultures. Representative developmental stage photos are of S. neumayeri reared at +2.6 °C and assorted pCO2 levels. Scale bar is 100 µm
Survivorship assays
Survivorship assays were used to assess whether development at elevated temperature and pCO2 would alter the tolerance of acute heat stress in EDSs of S. neumayeri. All EDSs survived temperatures that greatly exceeded their average habitat temperature of −1.9 °C. In general, survivorship curves look similar across all temperature and CO2 treatments. Survivorship following acute temperature exposure was high up to a tipping-point temperature (TT) beyond which it declined rapidly (Fig. 3).
Percent survivorship, following a 1 h acute heat stress and ~20 h recovery at culture temperatures, of four early developmental stages (a, e blastula; b, f gastrula; c, g prism; d, h 4-arm pluteus) of Sterechinus neumayeri reared at −0.7 °C (a–d) and +2.6 °C (e–h) under control pCO2 (~400 µatm, black squares and regression line) and elevated pCO2 (~650 µatm, gray circles and regression line; ~1,000 µatm, white triangles and perforated regression line) conditions. Symbols represent individual replicates (n = 100). Asterisk denotes significant pCO2 effect
S. neumayeri EDSs reared at −0.7 °C were extremely robust to pCO2, and no pCO2 effect was observed in the survivorship assays (Fig. 3a–d; Table 4). In contrast, negative pCO2 effects were observed for two warm culture EDSs, suggesting that multiple stressors are more detrimental than single stressors (Fig. 3e–h; Table 4). First, high pCO2 (>1,000 µatm) reduced thermal tolerance of warm culture blastulae at temperatures beyond the TT of 15 °C (p = 0.0005, Fig. 3e). This trend was also observed for blastulae reared at −0.7 °C but was not significant (p = 0.21). Second, medium pCO2 (650 µatm) reduced thermal tolerance of prism larvae reared at +2.6 °C (p = 0.0476, Fig. 3g).
Finally, due to genotypic and potential sampling differences between the cultures, direct comparisons based on culture temperature should be interpreted with caution. However, based on TTs, there appears to be no strong effect of culture temperature on the thermal tolerance of S. neumayeri EDSs. In both the cold and warm culture, blastulae were less thermotolerant than post-blastula stages by ~5 °C. Blastulae exhibited a TT of 15 °C. Post-blastula stages in both the cold and warm culture survived acute exposure up to 20 °C, with the exception of warm culture gastrulae which exhibited a TT of 18 °C (Fig. 4).
Summary of salient finding for the physiological toll of exposure to future ocean scenarios during early development of Sterechinus neumayeri, as assessed by a 1 h heat stress survivorship assay at four developmental stages. S. neumayeri embryos and larvae exhibited high tipping-point temperatures independent of development at −0.7 °C and +2.6 °C. Elevated pCO2 had an overall slight negative impact on blastulae and prisms reared at +2.6 °C. Scale bar is 100 µm
Discussion
The aim of this study was to assess the current physiological plasticity of the Antarctic sea urchin S. neumayeri in light of ocean warming and acidification and in the context of current ocean conditions. Here, we report two salient findings. First, as measured by autonomous pH sensors, we found that S. neumayeri EDSs currently experience extremely stable abiotic conditions in McMurdo Sound during the austral spring. Second, we found a remarkably high acute temperature tolerance of S. neumayeri EDSs reared at elevated pCO2 and temperature. Although in general, Antarctic organisms are predicted to be sensitive to environmental change (Peck 2005; Somero 2012), we show that S. neumayeri EDSs may be more physiologically tolerant of future conditions than previously thought (Byrne et al. 2013).
Field pH measurements
Measurements of ocean temperature and pH at Cape Evans, McMurdo Sound, Antarctica, during two seasons (austral spring 2011, 2012) were extremely stable and match previously documented environmental stability in this region (Littlepage 1965; Matson et al. 2011). For example, in 2010, pH ranged from 8.002 to 8.050 from 26 October to 15 November (Matson et al. 2011). Collectively, median pH at Cape Evans over three consecutive austral spring seasons was 8.019 (2010, Matson et al. 2011), 8.005 (2011, Matson 2012), and 8.016 (2012, this study). Relative to other ecosystems, pH data from Cape Evans show extremely stable inter-annual pH conditions. By contrast, pH in temperate and tropical reefs can vary as much as 0.544 and 0.253 pH units over only 30 days (Hofmann et al. 2011).
The period of stable pH at Cape Evans coincides with the spawning season of S. neumayeri (Pearse and Giese 1966; Brey et al. 1995). Previous plankton tows throughout McMurdo Sound show the presence of blastulae and gastrulae from November to December (Bosch et al. 1987). Thus, depending on local retention time post-fertilization, it is likely that EDSs from the Cape Evans S. neumayeri spawning population consistently develop at seawater pH of 8.0–8.1 and temperature of approximately −1.9 °C. Future ocean acidification and carbonate ion undersaturation, such as the conditions used in the experimental portion of this study, will likely be unprecedented for S. neumayeri in the coming decades.
Survivorship assays of S. neumayeri EDSs
Here, we report the changes in survivorship following a 1 h acute heat stress of S. neumayeri EDSs reared at elevated temperature and pCO2 that mimic predicted anthropogenic changes in the Southern Ocean. We hypothesized that development of S. neumayeri EDSs under seawater conditions outside their current ambient range would incur a physiological cost, such that tolerance of a secondary stressor would be reduced. We measured this cost by assessing tolerance of acute heat stress (Terblanche et al. 2011). We found negative pCO2 effects on acute thermal tolerance only when S. neumayeri were reared under elevated temperature of +2.6 °C (Fig. 4). While these results suggest a trade-off of development under a multi-stressor scenario and ability to cope with a secondary stress, the effect is relatively small from an ecological perspective. We therefore conclude that the effect of the multi-stressor scenario (ocean acidification and ocean warming together) on acute thermal tolerance is minor. S. neumayeri may be one of the more tolerant Antarctic marine invertebrates, exhibiting high physiological plasticity despite living under extremely stable conditions.
However, future multi-stressor scenarios may impact other physiological processes and long-term tolerances. For example, in recent research, S. neumayeri fertilization and early cleavage were negatively impacted by temperature (+1.5 and +3 °C) at a CO2 concentration of 1,370 ppm (pH 7.5, Ericson et al. 2012). Inter-individual variation observed in S. neumayeri fertilization success under elevated pCO2 may ameliorate such effects in the wild (Sewell et al. 2014). Furthermore, previous studies show compromised growth of S. neumayeri larvae reared at elevated pCO2 (Byrne et al. 2013; Yu et al. 2013). However, warming may increase larval growth (Byrne 2011). Byrne et al. (2013) showed that the combined effects of warming and CO2-acidification resulted in highly altered S. neumayeri larval body morphology and asymmetry, suggesting that there is a significant physiological effect of multi-stressor scenarios on the development of this polar echinoderm. Byrne et al. (2013) also found that under a multi-stressor scenario of temperature (+1 °C) and pCO2 (1,355 µatm, pH 7.6), development up to the gastrula stage was not affected but was negatively affected at the prism and pluteus stages with up to 83 percent of plutei showing abnormal development. Our findings show that these negative effects do not translate to secondary physiological trade-offs such that other functional traits, namely acute thermal tolerance, are compromised. Negative effects of warming and acidification on metamorphosis, juvenile growth and reproduction may swamp the small benefit of high physiological plasticity at the EDSs, although these have not yet been evaluated for S. neumayeri.
While normal development was not quantified in this study, culture temperature of +2.6 °C may have resulted in greater mortality of abnormal larvae such that the EDSs sampled in this study were selectively more thermotolerant than those observed by Byrne et al. (2013), which were reared at +1 °C. This type of outcome was observed in temperate purple sea urchin larvae, Strongylocentrotus purpuratus, where different genotypes were selected during culturing in various experimental future ocean acidification conditions (Pespeni et al. 2013). Ultimately, we assessed the embryos and larvae that survived culture conditions long enough to be sampled. For example, the pCO2 effect observed at the prism stage is largely due to the presence of abnormal larvae in the medium CO2 treatment (analysis not shown), and such larvae may not have survived to the pluteus stage. It should be noted that this treatment group was exposed to low pCO2 due to valve failures following sampling of the prism stage and higher survivorship of the pluteus stage could thus still be a factor of low pCO2 exposure. Other studies on sea urchin species show impaired development at only a few degrees above ambient conditions (Sewell and Young 1999; Byrne et al. 2009; Sheppard Brennand et al. 2010; Delorme and Sewell 2013). It is likely that a culture temperature of +2.6 °C is near the upper temperature limit of S. neumayeri early development.
Within species, developmental stages respond differently to abiotic stressors. While all S. neumayeri EDSs survived temperatures that greatly exceed their average habitat temperature of −1.9 °C, blastulae appear to be the most sensitive stage to abiotic stressors. Blastulae reared at −0.7 °C exhibited a pattern of reduced acute thermal tolerance with increasing pCO2. This pattern was statistically significant when blastulae were reared at +2.6 °C, suggesting that high pCO2 affects the performance of blastulae. Additionally, in both the cold and warm culture, thermal tolerance increased as much as 5 °C from 15 to 20 °C from blastula stage to gastrula, prism and pluteus stages, respectively. Sensitivity of this EDS has been observed previously where developmental progression of S. neumayeri between −0.8 and 0 °C was only affected by high pCO2 at the blastula stage (Yu et al. 2013).
In general, blastulae are one of the more sensitive developmental stages in echinoderms, especially with respect to temperature (Roccheri et al. 1986; Sconzo et al. 1995; Giudice et al. 1999). Large increases in gene expression are associated with the transition from hatched blastula to gastrula and later stages (Giudice et al. 1968; Howard-Ashby et al. 2006). Such genes include, for example, genes coding for heat shock proteins, which can increase thermal tolerance of post-blastula stages (Roccheri et al. 1986; Sconzo et al. 1995; Giudice et al. 1999). It is interesting that increased thermal tolerance coincides with increased pCO2 tolerance. Lower sensitivity of S. neumayeri post-blastula stages to pCO2 and acute temperature stress may be related to alterations in the larval transcriptome. Such changes in gene expression may allow the organism to overcome the negative effects of pCO2, demonstrating a link between gene expression and physiological plasticity that leads to tolerance of CO2 acidification (Evans et al. 2013). Thus, S. neumayeri may already harbor some genetic plasticity that allow EDSs to tolerate future ocean change, at least to some degree.
Temperate sea urchin gastrulae of S. purpuratus exhibit large changes in gene expression relative to control conditions (pH 8.1/435 μatm pCO2) when reared at naturally occuring levels of low pH (pH 7.77/813 μatm pCO2) but not at pH below natural exposures (pH 7.59/1,255 μatm pCO2). This suggests that gastrulae can compensate for pCO2 stress within the limits of current pH variability (Evans et al. 2013). Given that S. neumayeri EDSs experience a more stable pH environment than its temperate cousins, it is therefore unexpected that this species shows such high trait-based tolerance to elevated temperature and pCO2. In association with this study, the transcriptomic response of these S. neumayeri EDSs is underway (Drs. G. Dilly and G.E. Hofmann unpublished results) and will be useful in elucidating the mechanisms that underlie the pCO2 tolerance of this polar species.
Comparisons to other species
EDSs among marine invertebrates are inconsistently sensitive to multi-stressor scenarios (Pörtner and Farrell 2008; Byrne 2011). For example, our results contrast with the response of invertebrate larvae of both abalone Haliotis rufescens (Zippay and Hofmann 2010a) and red sea urchin Strongylocentrotus franciscanus (O’Donnell et al. 2008), which exhibit reduced thermal tolerance following development in CO2-acidified seawater. Many studies report other deleterious impacts of CO2 and temperature on EDSs stages of benthic marine invertebrates (Findlay et al. 2009, 2010; Parker et al. 2009, 2010; Sheppard Brennand et al. 2010; Anlauf et al. 2011; Byrne et al. 2011). For example, Anlauf et al. (2011) found that the combined effect of elevated pCO2 and temperature had a greater negative effect on growth of the coral Porites panamensis primary polyps than pCO2 alone. Findlay et al. (2010) also found that the post-larval growth rate of the barnacle Semibalanus balanoides was negatively impacted by reduced pH with a nonsignificant trend of further reduction in growth rate with increasing temperature of +4 °C. Parker et al. (2009) found reduced successful development of D-veliger larvae of oyster Saccostrea glomerata at high temperature and low pH treatments, compared to optimal temperature treatments. These studies highlight the importance of species-specific effects when attempting to predict biological changes to future multi-stressor scenarios, using measures of current physiological plasticity.
S. neumayeri temperature tolerance in context
Successful development of S. neumayeri nonfeeding EDSs at +2.6 °C and survival of acute exposure >20 °C above habitat temperatures is surprising for two reasons. First, Antarctic organisms are categorized as stenothermal due to evolutionary adaptation to the thermal stability of the Southern Ocean over evolutionary timescales (Peck 2005; Somero 2012). Second, gamete maturation in S. neumayeri occurs over the course of 12 to up to 24 months (Brockington et al. 2007) during which temperatures vary on an annual cycle of approximately −1.9 to −0.5 °C (Hunt et al. 2003).
Although longer temperature exposures will likely reduce upper temperature limits (Peck et al. 2009), the high acute temperature tolerance of S. neumayeri matches that of EDSs of eurythermal temperate species (Hammond and Hofmann 2010; Zippay and Hofmann 2010a, b). For example, gastrulae and 4-arm echinoplutei of temperate sea urchin S. purpuratus exhibit 50 percent survivorship following 1 h heat stress between 30 and 31 °C, a value up to 20 °C above average habitat temperatures (Hammond and Hofmann 2010). S. neumayeri are unlikely to ever experience temperatures up to 20 °C; however, assessing biological response to rapid warming can provide valuable insight into a species’ overall physiological tolerance and impacts on ecology (Terblanche et al. 2011).
High thermal tolerance of S. neumayeri pelagic EDSs may be an ancestral trait, stemming prior to speciation (Díaz et al. 2011) that followed the opening of the Drake Passage and establishment of the Antarctic Circumpolar Current (Lee et al. 2004). Such trait conservation may contribute to S. neumayeri’s extensive biogeographic range including colonization of warmer Antarctic archipelagos (Barnes et al. 2010). Our findings support the hypothesis that may be one of the least stenothermal Antarctic marine ectotherms (Barnes et al. 2010).
While S. neumayeri may be highly tolerant of acute temperature stress, this tolerance does not appear to be physiologically adaptive. In other words, development at +3 °C above −0.7 °C did not shift TTs by the same magnitude. This has been shown for adult S. neumayeri as well where a 60-day exposure of adult sea urchins to +3 °C did not affect acute upper temperature limits, which were approximately 15 °C (Peck et al. 2010). The lethal temperatures reported here and in Peck et al. (2010) are much higher than current habitat temperatures and ecologically irrelevant for predictions of warming in the Southern Ocean. However, these data support the hypothesis that while S. neumayeri is less stenothermal than previously thought (Barnes et al. 2010), its physiological adaptive capacity is limited (Peck 2005; Somero 2012).
Conclusion
We show that present-day S. neumayeri embryos and larvae are resilient to relatively large short-term multi-stressor scenarios. Other studies show variable sensitivities of physiological measures in S. neumayeri EDSs (i.e., development, growth, and asymmetry), and it remains unclear how ocean acidification and warming will ultimately affect the life cycle of this species. These experimental results also point in the direction of a need to better understand adaptation and genetics in response to ocean acidification (Evans and Hofmann 2012; Kelly and Hofmann 2012). As the ocean changes, new selection forces will act on the existing genetic structure. Individuals will be exposed to slowly changing environments which could enhance benefits of existing physiological plasticity, such as those described here, through maternal effects. It is significant that some studies show that traits of resilience are heritable (Sunday et al. 2011; Kelly et al. 2013) and local adaptation likely plays a strong role (Pespeni et al. 2013; Schaum et al. 2013). A high degree of present-day physiological plasticity and genetic variability may facilitate adaptation and long-term tolerance of future conditions. Given the predictions of rapid changes in oceans (IPCC 2007), identifying resilient and vulnerable species and variably tolerant populations is crucial in order to understand ecological impacts of ocean change.
References
Anlauf H, D’Croz L, O’Dea A (2011) A corrosive concoction: the combined effects of ocean warming and acidification on the early growth of a stony coral are multiplicative. J Exp Mar Biol and Ecol 397:13–20. doi:10.1016/j.jembe.2010.11.009
Barnes DKA, Peck LS, Morley SA (2010) Ecological relevance of laboratory determined temperature limits: colonization potential, biogeography and resilience of Antarctic invertebrates to environmental change. Glob Chang Biol 16:3164–3169. doi:10.1111/j.1365-2486.2010.02176.x
Bednaršek N, Tarling GA, Bakker DC, Fielding S, Cohen A, Kuzirian A, McCorkle D, Lézé B, Montagna R (2012) Description and quantification of pteropod shell dissolution: a sensitive bioindicator of ocean acidification. Glob Chang Biol 18:2378–2388
Bosch I, Beauchamp KA, Steele ME, Pearse JS (1987) Development, metamorphosis, and seasonal abundance of embryos and larvae of the Antarctic sea urchin Sterechinus neumayeri. Biol Bull 173:126–135. doi:10.2307/1541867
Boyd PW (2011) Beyond ocean acidification. Nat Geosci 4(5):273–274. doi:10.1038/ngeo1150
Brey T, Pearse J, Basch L, McClintock J, Slattery M (1995) Growth and production of Sterechinus neumayeri (Echinoidea: Echinodermata) in McMurdo sound, Antarctica. Mar Biol 124:279–292. doi:10.1007/bf00347132
Brockington S, Peck LS, Tyler PA (2007) Gametogenesis and gonad mass cycles in the common circumpolar Antarctic echinoid Sterechinus neumayeri. Mar Ecol Prog Ser 330:139–147
Buckley LB, Kingsolver JG (2012) Functional and phylogenetic approaches to forecasting species’ responses to climate change. Ann Rev Ecol Evol Syst 43:205–226
Byrne M (2011) Impact of ocean warming and ocean acidification on marine invertebrate life history stages: vulnerabilities and potential for persistence in a changing ocean. Oceanogr Mar Biol: Ann Rev 49:1–42
Byrne M, Ho M, Selvakumaraswamy P, Nguyen HD, Dworjanyn SA, Davis AR (2009) Temperature, but not pH, compromises sea urchin fertilization and early development under near-future climate change scenarios. Proc R Soc B: Biol Sci 276:1883–1888. doi:10.1098/rspb.2008.1935
Byrne M, Ho M, Wong E, Soars NA, Selvakumaraswamy P, Shepard-Brennand H, Dworjanyn SA, Davis AR (2011) Unshelled abalone and corrupted urchins: development of marine calcifiers in a changing ocean. Proc R Soc B: Biol Sci 278:2376–2383. doi:10.1098/rspb.2010.2404
Byrne M, Ho M, Koleits L, Price C, King C, Virtue P, Tilbrook B, Lamare M (2013) Vulnerability of the calcifying larval stage of the Antarctic sea urchin Sterechinus neumayeri to near-future ocean acidification and warming. Glob Chang Biol 19:2264–2275
Chown SL (2012) Trait-based approaches to conservation physiology: forecasting environmental change risks from the bottom up. Philos Trans R Soc B: Biol Sci 367:1615–1627
Chown SL, Gaston KJ (2008) Macrophysiology for a changing world. Proc R Soc B: Biol Sci 275:1469–1478
Clark MS, Peck LS (2009) HSP70 heat shock proteins and environmental stress in Antarctic marine organisms: a mini-review. Mar Genom 2:11–18
Clark D, Lamare M, Barker M (2009) Response of sea urchin pluteus larvae (Echinodermata: Echinoidea) to reduced seawater pH: a comparison among a tropical, temperate, and a polar species. Mar Biol 156:1125–1137
Dawson TP, Jackson ST, House JI, Prentice IC, Mace GM (2011) Beyond predictions: biodiversity conservation in a changing climate. Science 332:53–58
Delorme NJ, Sewell MA (2013) Temperature limits to early development of the New Zealand sea urchin Evechinus chloroticus (Valenciennes, 1846). J Thermal Biol 38:218–224
Díaz A, Féral J-P, David B, Saucède T, Poulin E (2011) Evolutionary pathways among shallow and deep-sea echinoids of the genus Sterechinus in the Southern Ocean. Deep-Sea Res Part II: Top Stud Oceanogr 58:205–211
Dickson AG, Millero FJ (1987) A comparison of the equilibrium constants for the dissociation of carbonic acid in seawater media. Deep-Sea Res Part A-Oceanogr Res Papers 34:1733–1743. doi:10.1016/0198-0149(87)90021-5
Dickson AG, Sabine CL, Christian JR (Eds) (2007) Guide to best practices for ocean CO2 measurements. PICES Special Publication 3, 191 pp
Dupont S, Thorndyke M (2009) Impact of CO2-driven ocean acidification on invertebrates early life-history—what we know, what we need to know and what we can do. Biogeosci Discuss 6:3109–3131
Enzor LA, Zippay ML, Place SP (2013) High latitude fish in a high CO2 world: synergistic effects of elevated temperature and carbon dioxide on the metabolic rates of Antarctic notothenioids. Comp Biochem Physiol Part A: Molec Integrat Physiol 164:154–161
Ericson JA, Ho MA, Miskelly A, King CK, Virtue P, Tilbrook B, Byrne M (2012) Combined effects of two ocean change stressors, warming and acidification, on fertilization and early development of the Antarctic echinoid Sterechinus neumayeri. Polar Biol 35:1027–1034. doi:10.1007/s00300-011-1150-7
Evans TG, Hofmann GE (2012) Defining the limits of physiological plasticity: how gene expression can assess and predict the consequences of ocean change. Philos Trans R Soc B: Biol Sci 367:1733–1745
Evans TG, Chan F, Menge BA, Hofmann GE (2013) Transcriptomic responses to ocean acidification in larval sea urchins from a naturally variable pH environment. Molec Ecol 22:1609–1625
Fabry VJ, McClintock JB, Mathis JT, Grebmeier JM (2009) Ocean acidification at high latitudes: the bellweather. Oceanography 22:160–171
Fangue NA, O’Donnell MJ, Sewell MA, Matson PG, MacPherson AC, Hofmann GE (2010) A laboratory-based, experimental system for the study of ocean acidification effects on marine invertebrate larvae. Limnol Oceanogr Meth 8:441–452. doi:10.4319/lom.2010.8.441
Feely RA (2004) Impact of anthropogenic CO2 on the CaCO3 system in the oceans. Science 305:362–366. doi:10.1126/science.1097329
Findlay HS, Kendall MA, Spicer JI, Widdicombe S (2009) Post-larval development of two intertidal barnacles at elevated CO2 and temperature. Mar Biol 157:725–735. doi:10.1007/s00227-009-1356-1
Findlay HS, Kendall MA, Spicer JI, Widdicombe S (2010) Relative influences of ocean acidification and temperature on intertidal barnacle post-larvae at the northern edge of their geographic distribution. Estuar Coast Shelf Sci 86:675–682. doi:10.1016/j.ecss.2009.11.036
Fyfe JC (2006) Southern Ocean warming due to human influence. Geophys Res Lett 33:L19701
Gienapp P, Teplitsky C, Alho J, Mills J, Merilä J (2008) Climate change and evolution: disentangling environmental and genetic responses. Molec Ecol 17:167–178
Gille ST (2002) Warming of the Southern Ocean since the 1950s. Science 295:1275–1277. doi:10.1126/science.1065863
Giudice G, Mutolo V, Donatuti G (1968) Gene expression in sea urchin development. Dev Genes Evol 161:118–128
Giudice G, Sconzo G, Roccheri MC (1999) Studies on heat shock proteins in sea urchin development. Dev Growth Differ 41:375–380
Hammond LM, Hofmann GE (2010) Thermal tolerance of Strongylocentrotus purpuratus early life history stages: mortality, stress-induced gene expression and biogeographic patterns. Mar Biol 157:2677–2687. doi:10.1007/s00227-010-1528-z
Harvey BP, Gwynn-Jones D, Moore PJ (2013) Meta-analysis reveals complex marine biological responses to the interactive effects of ocean acidification and warming. Ecol Evol 3:1016–1030. doi:10.1002/ece3.516
Hoffmann AA, Sgrò CM (2011) Climate change and evolutionary adaptation. Nature 470:479–485
Hofmann GE, Buckley BA, Airaksinen S, Keen JE, Somero GN (2000) Heat-shock protein expression is absent in the Antarctic fish Trematomus bernacchii (family Nototheniidae). J Exp Biol 203:2331–2339
Hofmann GE, Lund SG, Place SP, Whitmer AC (2005) Some like it hot, some like it cold: the heat shock response is found in New Zealand but not Antarctic notothenioid fishes. J Exp Mar Biol Ecol 316:79–89
Hofmann GE, Smith JE, Johnson KS, Send U, Levin LA, Micheli F, Paytan A, Price NN, Peterson B, Takeshita Y, Matson PG, Crook ED, Kroeker KJ, Gambi MC, Rivest EB, Frieder CA, Yu PC, Martz TR (2011) High-frequency dynamics of ocean pH: a multi-ecosystem comparison. PLoS One 6:e28983. doi:10.1371/journal.pone.0028983
Hönisch B, Ridgwell A, Schmidt DN, Thomas E, Gibbs SJ, Sluijs A, Zeebe R, Kump L, Martindale RC, Greene SE, Kiessling W, Ries J, Zachos JC, Royer DL, Barker S, Marchitto TM Jr, Moyer R, Pelejero C, Ziveri P, Foster GL, Williams B (2012) The geological record of ocean acidification. Science 335:1058–1063. doi:10.1126/science.1208277
Howard-Ashby M, Materna SC, Brown CT, Tu Q, Oliveri P, Cameron RA, Davidson EH (2006) High regulatory gene use in sea urchin embryogenesis: implications for bilaterian development and evolution. Dev Biol 300:27–34
Hunt BM, Hoefling K, Cheng CC (2003) Annual warming episodes in seawater temperatures in McMurdo Sound in relationship to endogenous ice in notothenioid fish. Antarct Sci 15:333–338
IPCC (2007) Contribution of working group II to the fourth assessment report of the intergovernmental panel on climate change. In: Parry ML, Canziani OF, Palutikof JP, van der Linden PJ, Hanson CE (eds) Cambridge University Press, Cambridge
Kelly MW, Hofmann GE (2012) Adaptation and the physiology of ocean acidification. Func Ecol 27:980–990. doi:10.1111/j.1365-2435.2012.02061.x
Kelly MW, Padilla-Gamiño JL, Hofmann GE (2013) Natural variation and the capacity to adapt to ocean acidification in the keystone sea urchin Strongylocentrotus purpuratus. Glob Chang Biol 19:2536–2546. doi:10.1111/gcb.12251
Kurihara H (2008) Effects of CO2-driven ocean acidification on the early developmental stages of invertebrates. Mar Ecol Prog Ser 373:275–284
Lee YH, Song M, Lee S, Leon R, Godoy SO, Canete I (2004) Molecular phylogeny and divergence time of the Antarctic sea urchin (Sterechinus neumayeri) in relation to the South American sea urchins. Antarct Sci 16:29–36
Littlepage JL (1965) Oceanographic investigations in McMurdo sound, Antarctica. Antarct Res Ser 5:1–37
Marcott SA, Shakun JD, Clark PU, Mix AC (2013) A reconstruction of regional and global temperature for the past 11,300 years. Science 339:1198–1201
Martz TR, Connery JG, Johnson KS (2010) Testing the Honeywell Durafet (R) for seawater pH applications. Limnol Oceanogr Meth 8:172–184. doi:10.4319/lom.2010.8.172
Matson PG (2012) Ocean acidification in nearshore marine ecosystems: natural dynamics of ocean pH variation and impacts on sea urchin larvae. Dissertation, University of California Santa Barbara
Matson PG, Martz TR, Hofmann GE (2011) High-frequency observations of pH under Antarctic sea ice in the southern Ross Sea. Antarct Sci 23:607–613. doi:10.1017/s0954102011000551
McClintock J, Ducklow H, Fraser W (2008) Ecological responses to climate change on the Antarctic Peninsula. Am Sci 96:302–310
McNeil BI, Matear RJ (2008) Southern Ocean acidification: a tipping point at 450-ppm atmospheric CO2. Proc Natl Acad Sci 105:18860–18864. doi:10.1073/pnas.0806318105
Mehrbach C, Culberso Ch, Hawley JE, Pytkowic Rm (1973) Measurement of apparent dissociation constants of carbonic acid in seawater at atmospheric pressure. Limnol Oceanogr 18:897–907
Montes-Hugo M, Doney SC, Ducklow HW, Fraser W, Martinson D, Stammerjohn SE, Schofield O (2009) Recent changes in phytoplankton communities associated with rapid regional climate change along the western Antarctic Peninsula. Science 323:1470–1473
Naveen R, Lynch HJ, Forrest S, Mueller T, Polito M (2012) First direct, site-wide penguin survey at Deception Island, Antarctica, suggests significant declines in breeding chinstrap penguins. Polar Biol 35:1879–1888
O’Donnell MJ, Hammond LM, Hofmann GE (2008) Predicted impact of ocean acidification on a marine invertebrate: elevated CO2 alters response to thermal stress in sea urchin larvae. Mar Biol 156:439–446. doi:10.1007/s00227-008-1097-6
Orr JC, Fabry VJ, Aumont O, Bopp L, Doney SC, Feely RA, Gnanadesikan A, Gruber N, Ishida A, Joos F, Key RM, Lindsay K, Maier-Reimer E, Matear R, Monfray P, Mouchet A, Najjar RG, Plattner G-K, Rodgers KB, Sabine CL, Sarmiento JL, Schlitzer R, Slater RD, Totterdell IJ, Weirig M-F, Yamanaka Y, Yool A (2005) Anthropogenic ocean acidification over the twenty-first century and its impact on calcifying organisms. Nature 437:681–686. doi:10.1038/nature04095
Parker LM, Ross PM, O’Connor WA (2009) The effect of ocean acidification and temperature on the fertilization and embryonic development of the Sydney rock oyster Saccostrea glomerata (Gould 1850). Glob Chang Biol 15:2123–2136. doi:10.1111/j.1365-2486.2009.01895.x
Parker LM, Ross PM, O’Connor WA (2010) Comparing the effect of elevated pCO2 and temperature on the fertilization and early development of two species of oysters. Mar Biol 157:2435–2452. doi:10.1007/s00227-010-1508-3
Parmesan C, Yohe G (2003) A globally coherent fingerprint of climate change impacts across natural systems. Nature 421:37–42
Pearse JS, Giese AC (1966) Food, reproduction and organic constitution of common Antarctic echinoid Sterechinus neumayeri (Meissner). Biol Bull 130:387–401. doi:10.2307/1539745
Peck LS (2005) Prospects for survival in the Southern Ocean: vulnerability of benthic species to temperature change. Antarct Sci 17:497–507. doi:10.1017/s0954102005002920
Peck LS, Clark MS, Morley SA, Massey A, Rossetti H (2009) Animal temperature limits and ecological relevance: effects of size, activity and rates of change. Func Ecol 23:248–256. doi:10.1111/j.1365-2435.2008.01537.x
Peck LS, Morley SA, Clark MS (2010) Poor acclimation capacities in Antarctic marine ectotherms. Mar Biol 157:2051–2059. doi:10.1007/s00227-010-1473-x
Pespeni MH, Sanford E, Gaylord B, Hill TM, Hosfelt JD, Jaris HK, LaVigne M, Lenz EA, Russell AD, Young MK (2013) Evolutionary change during experimental ocean acidification. Proc Natl Acad Sci 110:6937–6942
Place SP, Zippay ML, Hofmann GE (2004) Constitutive roles for inducible genes: evidence for the alteration in expression of the inducible hsp70 gene in Antarctic notothenioid fishes. Am J Physiol Regul Integr Comp Physiol 287:R429–R436. doi:10.1152/ajpregu.00223.2004
Pörtner HO, Farrell AP (2008) Physiology and climate change. Science 322:690–692. doi:10.1126/science.1163156
Pörtner HO, Peck L, Somero G (2007) Thermal limits and adaptation in marine Antarctic ectotherms: an integrative view. Philos Trans R Soc B: Biol Sci 362:2233–2258. doi:10.1098/rstb.2006.1947
Pörtner HO, Peck LS, Somero GN (2012) Mechanisms defining thermal limits and adaptation in marine ectotherms: an integrative view. Antarctic ecosystems: an extreme environment in a changing world. Wiley, Chichester. doi:10.1002/9781444347241.ch13
Robbins LL, Hansen ME, Kleypas JA, Meylan SC (2010) CO2calc—a user-friendly seawater carbon calculator for Windows, Max OS X, and iOS (iPhone). U.S. Geological Survey Open-File Report 2010–1280
Roccheri MC, Sconzo G, Larosa M, Oliva D, Abrignani A, Giudice G (1986) Response to heat shock of different sea urchin species. Cell Differ 18:131–135. doi:10.1016/0045-6039(86)90007-2
Schaum E, Rost B, Millar AJ, Collins S (2013) Variation in plastic responses to ocean acidification in a globally distributed picoplankton species. Nat Clim Chang 3:298–302
Sconzo G, Ferraro MG, Amore G, Giudice G, Cascino D, Scardina G (1995) Activation by heat shock of hsp70 gene transcription in sea urchin embryos. Biochem Biophys Res Commun 217:1032–1038. doi:10.1006/bbrc.1995.2873
Sewell MA, Hofmann GE (2011) Antarctic echinoids and climate change: a major impact on the brooding forms. Glob Change Biol 17:734–744
Sewell MA, Young CM (1999) Temperature limits to fertilization and early development in the tropical sea urchin Echinometra lucunter. J Exp Mar Biol Ecol 236:291–305. doi:10.1016/s0022-0981(98)00210-x
Sewell MA, Millar RB, Yu PC, Kapsenberg L, Hofmann GE (2014) Ocean acidification and fertilization in the Antarctic sea urchin Sterechinus neumayeri: the importance of polyspermy. Environ Sci Technol 48:713–722
Sheppard Brennand H, Soars N, Dworjanyn SA, Davis AR, Byrne M (2010) Impact of ocean warming and ocean acidification on larval development and calcification in the sea urchin Tripneustes gratilla. PLoS One 5:e11372. doi:10.1371/journal.pone.0011372
Somero GN (2012) The physiology of global change: linking patterns to mechanisms. Ann Rev Mar Sci 4:39–61
Stammerjohn SE, Martinson DG, Smith RC, Iannuzzi RA (2008) Sea ice in the western Antarctic Peninsula region: spatio-temporal variability from ecological and climate change perspectives. Deep-Sea Res Part II: Top Stud Oceanogr 55:2041–2058
Stanwell-Smith D, Peck LS (1998) Temperature and embryonic development in relation to spawning and field occurrence of larvae of three Antarctic echinoderms. Biol Bull 194:44–52. doi:10.2307/1542512
Steinberg DK, Martinson DG, Costa DP (2012) Two decades of pelagic ecology in the Western Antarctic Peninsula. Oceanography 25:56–67
Sunday JM, Crim RN, Harley CDG, Hart MW (2011) Quantifying rates of evolutionary adaptation in response to ocean acidification. PLoS One 6:e22881. doi:10.1371/journal.pone.0022881
Sunday JM, Bates AE, Dulvy NK (2012) Thermal tolerance and the global redistribution of animals. Nat Clim Chang 2:686–690
Takahashi T, Sutherland SC, Wanninkhof R, Sweeney C, Feely RA, Chipman DW, Hales B, Friederich G, Chavez F, Sabine C et al (2009) Climatological mean and decadal change in surface ocean pCO2, and net sea-air CO2 flux over the global oceans. Deep-Sea Res Part II: Top Stud Oceanogr 56:554–577
Terblanche JS, Hoffmann AA, Mitchell KA, Rako L, le Roux PC, Chown SL (2011) Ecologically relevant measures of tolerance to potentially lethal temperatures. J Exp Biol 214:3713–3725
Tomanek L (2010) Variation in the heat shock response and its implication for predicting the effect of global climate change on species’ biogeographical distribution ranges and metabolic costs. J Exp Biol 213:971–979
Turley C, Eby M, Ridgwell AJ, Schmidt DN, Findlay HS, Brownlee C, Riebesell U, Fabry VJ, Feely RA, Gattuso JP (2010) The societal challenge of ocean acidification. Mar Pollut Bull 60:787–792. doi:10.1016/j.marpolbul.2010.05.006
Visser ME (2008) Keeping up with a warming world; assessing the rate of adaptation to climate change. Proc R Soc B: Biol Sci 275:649–659
Yu PC, Sewell MA, Matson PG, Rivest EB, Kapsenberg L, Hofmann GE (2013) Growth attenuation with developmental schedule progression in embryos and early larvae of Sterechinus neumayeri raised under elevated CO2. PLoS One 8:e52448
Zippay ML, Hofmann GE (2010a) Effect of pH on gene expression and thermal tolerance of early life history stages of Red Abalone (Haliotis rufescens). J Shellfish Res 29:429–439. doi:10.2983/035.029.0220
Zippay ML, Hofmann GE (2010b) Physiological tolerances across latitudes: thermal sensitivity of larval marine snails (Nucella spp.). Mar Biol 157:707–714
Acknowledgments
The authors would like to thank members of the US Antarctic Program: R. Robbins and S. Rupp for sea urchin collections and sensor deployments and Crary Laboratory staff of 2011 and 2012 for resource support at McMurdo Station, Antarctica. We thank Professor W. Rice for advice on statistical analysis. Additionally, we especially thank Dr. P.C. Yu and other members of the Bravo-134 field team for their support during larval culture and water chemistry measurements (Professor M. Sewell, Dr. G. Dilly, E. Hunter, Dr. A. Kelley, Dr. P. Matson, E. Rivest, and O. Turnross). We are grateful for the comments from two anonymous reviewers that led to the improvement of this manuscript. This research was supported by US National Science Foundation (NSF) Grant ANT-0944201 to GEH. L.K. was supported by a NSF Graduate Research Fellowship.
Author information
Authors and Affiliations
Corresponding author
Rights and permissions
About this article
Cite this article
Kapsenberg, L., Hofmann, G.E. Signals of resilience to ocean change: high thermal tolerance of early stage Antarctic sea urchins (Sterechinus neumayeri) reared under present-day and future pCO2 and temperature. Polar Biol 37, 967–980 (2014). https://doi.org/10.1007/s00300-014-1494-x
Received:
Revised:
Accepted:
Published:
Issue Date:
DOI: https://doi.org/10.1007/s00300-014-1494-x