Abstract
A long-term study on the maturity cycle of Antarctic krill was conducted in a research aquarium. Antarctic krill were either kept individually or in groups for 8 months under different temperature and food conditions, and the succession of female maturity stages and intermoult periods were observed. In all cases regression and re-maturation of external sexual characteristics were observed, but there were differences in length of the cycle and intermoult periods between the experimental conditions. Based on these results, and information available from previous studies, we suggest a conceptual model describing seasonal cycle of krill physiology which provides a framework for future studies and highlight the importance of its link to the timings of the environmental conditions.
Similar content being viewed by others
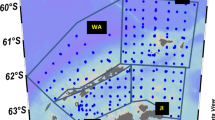
Avoid common mistakes on your manuscript.
Introduction
Antarctic krill (Euphausia superba) are known to be more productive and occur at higher density in the Southwest Atlantic compared to other areas within their distributional range (Marr 1962; Mackintosh 1973). Winter sea-ice extent is thought to provide winter feeding habitat and subsequent spring bloom, which could be the key determinants for annual krill abundance and krill recruitment (Siegel and Loeb 1995). An extensive data analysis further suggested that primary production may be the key parameter determining krill abundance in Southwest Atlantic (Atkinson et al. 2004). On the other hand Reid (2001) hypothesized high krill abundance in this area is due to warmer water temperatures, longer summers, as well as higher annual primary production in the Southwest Atlantic, however, the relationship is not simple and there are both annual and regional exceptions (Constable et al. 2003; Smetacek and Nicol 2005).
The life history of Antarctic krill is thought to be very flexible, and this is regarded as one of their main strategies to make use of favorable conditions for their growth and reproduction when ever they can. Crustacean reproduction and growth (moulting) are generally closely linked and scheduled by various cues from the environment (Nelson 1991). To date, there is no unifying concept of how these environmental features are linked to Antarctic krill reproductive ecology.
A series of intensive experimental studies were done on krill maturity in 1970s–1980s (e.g. Makarov 1975; Denys and McWhinnie 1982; Poleck and Denys 1982; Ikeda 1985; Thomas and Ikeda 1987). They observed the maturity stages undergoing a cycle where external sexual characteristics reduced from fully mature to juvenile, then once again developed to full maturity under conditions of constant temperature and food in complete darkness. They suggested that this cyclic event was controlled by an endogenous rhythm rather than being cued by environmental factors (Thomas and Ikeda 1987). Later, Hirano et al. (2003) succeeded, for the first time, in breeding krill in captivity and they implicated exogenous environmental factors. They induced krill reproduction by using photoperiod as a cue, and concluded photoperiod is one of the most important factors dictating the onset of krill maturity (Port of Nagoya public aquarium 2002; Hirano et al. 2003). Krill appear to shift their food from phytoplankton to grazing on ice algae during winter (e.g. Marschall 1988), and therefore dense winter sea-ice concentrations are also known to promote early female gonadal development and spawning (Siegel and Loeb 1995). Cuzin-Roudy and Buchholz (1999) proposed a conceptual model of ovarian maturation and spawning in relation to the moult cycle for Northern krill (Meganyctiphanes norvegica), which is considered to be operating in a similar manner for Antarctic krill (Cuzin-Roudy 2000).
The purpose of this current study was to examine the possible effects of food, temperature, and photoperiod on krill maturity by using a long-term experiment and by revisiting experimental studies previously reported. We further propose a new way of looking at how seasonal maturity and reproduction are governed in the natural environment.
Materials and methods
Live Antarctic krill were collected using a rectangular midwater trawl net (Baker et al. 1973) near Davis station on 5 February 2004 (65°23′S, 80°13′E) by RSV Aurora Australis. Krill were evenly distributed in seven 200–250 l tanks in cold laboratories and seawater was continuously supplied using the ship’s clean experimental seawater system whilst the vessel was south of polar front. Once the seawater temperature exceeded 2.5°C, the continuous water supply was closed and 50% of the each tank water was manually replaced by chilled seawater (0.5°C) each day. Krill were kept in the dark until their return to the Australian Government Antarctic Division’s Kingston headquarters on 13 February 2004.
After their return, krill were transferred to 2,000 l tank and acclimated to the aquarium conditions (King et al. 2003). This population was kept under dark condition at 0.5°C and was used as the stock population (SP) of the whole experiment.
Individual experiments
A sample of individual krill (n = 26) were isolated in flow-through 5-l jars and were maintained in darkness. Water was circulated between the jars and a 200 l sump which had bio-filters installed to clean the water. The first series of experiments started on 1 April (I–1C–F and I–3C–F), and were kept under two different temperature conditions (1 and 3°C) for up to 288 days. Each jar was fed with 30 ml of Phaeodactylum culture per week. The second series of experiments started from 28 April (I–4C–WF) or 24May (I–4C–S). All jars in this series were kept in 4°C but under different feeding regimes, with 30 ml of Phaeodactylum sp. three times a week for I–4C–WF, whereas series I–4C–S was starved for the whole period. The water flow was closed for 24 h on the days of feeding to maximize their food intake. Each morning all jars were checked for moults, and if moults were observed they were put in 10% formalin seawater for later analysis.
Group experiment
An experiment was set up with three groups of 80 individuals in 200-l tanks with different feeding regimes. Series G–0–AF were fed phytoplankton and animal source food, series G–0–PF were fed only phytoplankton, and series G–0–S were not fed. Although no food was given to series G–0–S, the entire tank system was set up in a single water circulation system, so we could not establish a completely food-free environment, and therefore they were exposed to a low level of food. The groups were kept in the dark until 12 July and then were changed to a 24 h light (1,000 lux) environment from 13 July onwards.
The stock population (SP) was used to supply the animals for each experimental series and this stock population was continually kept in a single 2,000 l tank following the animals’ transfer to the AAD aquarium in February. The remaining animals were continuously kept under conditions of complete darkness until the end of all the experiments.
Maturity staging was undertaken following Bargmann (1945). The reason of employing this system rather than often used Makarov and Denys (1981) was because Bargmann’s system allows a better description of the regression of external sexual characteristics of female krill. Table 2 summarizes the staging system in relation to ovary status, and also the maturity scores that we used to in this study to calculate average maturation for each experimental series. Higher values represent greater maturity, lower values indicate immaturity.
Results
Figure 1 shows mean maturity scores (±SE) of the individual experiments plotted against the number of moulting events since the start of the experiment. The individual experiments at 4°C (I–4C–WF and I–4C–S) were separated from the stock population (Series-SP) later than those in individuals at lower temperatures (I–1C–F and I–3C–F), so were likely to have been through one (I–4C–WF) or two (I–4C–S) moulting events whilst still in the stock population. Since the other two jar experiments started on the 1st of April, their moulting events are plotted from the 2nd or 3rd moults, respectively.
Figure 2 shows the chronological succession of mean maturity scores of each series of individual experiments (Series-I), as well as the maturity progression of krill held in groups (Series-G), and in the stock population (Series-SP) which all the krill used in the experiments were supplied from. The group series were light-treated from the 13th of July. The maturity stages of the group and stock population series were only reported from September onwards.
Chronological succession of mean maturity scores (±SD) of each experimental series of individual experiments (I series), krill held in groups (G series), and the stock population (SP series). The G-series were light treated from the 13th of July. The maturity stages of G and SP series were observed from August onwards
There was a general cyclic pattern in the maturity succession for both experimental series. The mean maturity scores were at the lowest during May (2nd–4th moulting events), advancing from June until September (5th–7th moulting events), peaking in October (8th–10th moulting events), then regressing thereafter. For the individual experiments, the higher temperature series (I–4C series especially I–4C–S) exhibited greater thelycum regression and later recovery compared to those in lower temperature regimes after once most regressed (Fig. 1).
Those kept for prolonged period (I–1C–F, I–3C–F) did not maintain their maturity but started to regress once they reached their peak of maturity. Not all of the krill reached Stage-E, some only reached Stage D before they started to regress (Figs. 1, 2).
For the group experiment, thelycum maturity was examined for the period after they were given light cues. The mean maturity scores fell within the range of standard deviations (SD) obtained in the individual experiments. No consistent patterns or differences in trends were observed between different feeding regimes amongst the group experiments. However, the stock population, which was kept dark throughout the experiment, showed the lowest maturity scores compared to the group experiments (except on one occasion) during the two-month period when maturity was monitored for these series (Fig. 2).
Figure 3 shows the changes in the mean intermoult period (IMP) against the number of moulting events. There was a general trend for the IMP to be shorter at the start of the experiment, to increase in the middle of the experiment then to decline thereafter. Interestingly the initial IMP increase depended on the temperature; the I–1C–F series showed an increase in IMP until the 6th moulting event, whereas the I–3C–F and I–4C–WF series only increased for the initial three moulting events. Higher temperature treatment resulted in overall shorter IMPs throughout. The only different pattern observed was for the starved series (I–4C–S), which showed an IMP increase until the 6th moulting event then showed a gradual decrease thereafter. The results of Thomas and Ikeda (1987) are also plotted on the same chart with the x-axis adjusted to make the comparison with our current study possible. Their results show a similar pattern to ours but the range of IMPs is smaller and their period of extended IMPs is longer.
Changes of mean intermoult periods (IMP) against the number of moulting events. Results from Thomas and Ikeda (1987) also plotted
Discussion
The general progression of the seasonal maturity cycle in our experiment agreed with that observed by Thomas and Ikeda (1987) showing winter regression followed by re-maturation, which also seems to be occurring in the wild as well (Siegel et al. 2004). Our results further indicated that the pattern and length for completion of the maturity cycle are also affected by the environmental conditions.
Although the sample size of our experiment was small, starved animals seems to take longer to start recovering their maturity compared to the better fed animals under same temperature regime (4°C) but they peak at around the same time (moult 8–9; Fig. 2). IMPs were longer in lower temperatures and the overall trend for the IMP was, short at the beginning, an increase towards the middle of the cycle, and then again a decrease towards the end of the cycle. A temperature-dependent difference in the timing at which the IMP started to decrease was observed; earlier in the higher temperature and later in the lower.
Because of the variable Antarctic environment, the temporal relationship between natural events such as ice retreat, coupled with food availability and temperature changes with the timings in the maturity cycle is likely to be very important for krill over-wintering and reproductive success. To date, we only have patchy information about the krill maturation cycles derived from a small number of field surveys and experiments (i.e. Makarov 1975; Poleck and Denys 1982; Thomas and Ikeda 1987; Siegel et al. 2004), and therefore further investigations are required to draw any firm conclusions about the entire krill maturation cycle. In the following sections we examine the possible effect of environmental factors on the maturity cycle inferred from our current study and previous studies, and finally suggest a working hypothesis to provide a framework for the future surveys, experiments, and modeling.
Maturity under controlled environments
Light condition
Our study generally matched the seasonal maturation cycle observed by Thomas and Ikeda (1987) indicating that light is not necessary for maturation in krill. This runs counter to observations by Hirano et al. (2003) who demonstrated that light can be an effective cue to accelerate maturity in female krill.
Given the observation that the thelycum development needs 5–6 moults from the most regressed form (Stage-B) to be morphologically ready for mating, it would not be possible for a regressed female (stage B) to mature and mate within 1–2 months as demonstrated in Hirano et al. (2003) assuming an IMP of 20–30 days. Hirano’s result can be explained by assuming that their krill which were matured by light cues were in fact individuals close to maturation. Indeed, we observed large error bars (SD) surrounding the seasonal cycle of the mean maturity scores (Fig. 2) which indicates that some krill in the population may always be in relatively advanced maturity stages. These individuals can mature and reproduce within relatively short period after appropriate cues. There are several plateaus in ovarian maturity which need additional resources or environmental cues for their further development (Ross and Quetin 2000). The maturity and spawning observed after light cues (Hirano et al. 2003) may have been of those individuals at advanced maturity plateaus, and the light cue may have accelerated further developments of their ovaries. However, it is still unclear in which process the light cue was effective. Although 24 h light conditions is very often the light regime of the peak spawning season, there is also a possibility that the effect of light cues in Hirano et al. (2003) is an irregular response due to an abrupt and unnatural change to 24 h light from complete darkness or an 8 h light period.
Once the external maturity of krill starts to regress, they then go through a complete cycle before re-maturing. The period from the start of regression until their re-maturation up to Stage-E (the stage ready to start previtellogenesis and vitellogenesis) requires at least 10 consecutive moulting events, and maybe more depending on their condition.
Overall, the contribution of light is unclear at this stage and to develop further understanding on environmental influences on krill reproduction it will be important to understand which process may be regulated by light conditions.
Food condition
The individual experiments showed that the well fed group (I–4C–WF) exhibited earlier recovery of maturity stage compared to the starved group (I–4C–S) at the same temperature setting (Fig. 1). This agrees with the findings of Thomas and Ikeda (1987), although similar results were not clearly seen in our group experiments due to the short period of maturity observation in these groups.
So far, no study has examined effect of quality and quantity of food on krill maturation and fecundity. In our experimental setup, the range of food quality was limited and therefore it is difficult to infer what the implications of seasonal and regional variation of food quality and quality are on the trends in the natural maturation cycle. Further detailed study dedicated to the effect of food quality on the process of the maturation is necessary.
Temperature
Temperature differences between the series of the individual experiments exhibited effects on both IMP trends across the period and the degree of maturity regression. The most striking difference in patterns of IMP between different temperature treatments was in the timing when the IMP reduces (Fig. 3). The IMP at 1°C started to decrease in September–October (from 7th moult) when the mean maturity score reached D-stage (4 in score), whereas animals maintained at higher temperatures (3 and 4°C) began to decrease in June (after 4th moults) which coincides with the time when they were starting to re-mature from the regressed stages. Since most of the mature females were spent when they were brought back to the laboratory in February, and they were kept at 0.5°C until the start of experiment in early April, they should have gone through 2–3 moulting events after they originally started to regress before the start of our experiment (Table 1). Therefore we can assume that they were in their 9–10th moult for 1°C, and 6–7th moult for 3 and 4°C when the decrease of their IMP occurred (Table 2).
Thomas and Ikeda (1987) showed a longer IMP during regression and this shortened from November to January (after 11–12th moulting events) after they externally matured and the ovaries started to develop prior to spawning. From our current study, it seems as if the timing of the increase and decrease in the IMP does not necessary coincide with the ovarian development but is affected by the temperature. Although temperature and feeding condition are known to affect the length and the pattern of the IMPs (Buchholz 1991; Kawaguchi et al. 2006), at this stage, we cannot explain why the IMP changes within a maturity cycle under constant temperature. Other than the seasonal temperature cycle in the environment, seasonal change of behaviour, especially the preference of krill for deeper water layers during winter (Jackowski 2002; Siegel 2005; Taki et al. 2005) may affect the environmental temperatures they experience.
Other possible explanations
An alternative approach to analyzing these results is to focus on the timings at the start of each of the individual experimental series. There appear to be time lags in the cycle depending on the timing of the start of the experiment i.e., the time at which the animals were subdivided from the stock population. The later they were subdivided, the more moulting events were needed to recover their maturity. Although there were variations in feeding conditions, because stock population was given essentially the same rations as individuals in the fed experiments [I–1C–F and I–3C–F (roughly twothird of I–4C–WF)] this may not be a realistic explanation. Temperature may have had some effect; however, given the fact that a similar pattern was observed for the jar series at 1 and 3°C, we would not expect much difference with 0.5°C in the stock population.
A further explanation may be the difference in energy expenditures between the individuals kept in small jars and those in the large holding tanks. Krill in large tanks would be expected to consume more energy by swimming around in a larger space, whereas krill kept in jars are observed to be relatively immobile most of the time. The phytoplankton food concentrations in our experiments were within the range of 0.25–0.3 μg C ml−1 (assuming 10 μg C/106 cells; Ikeda and Thomas 1987) or 5–6 μg Chl l−1 (assuming C/Chl = 50), which was higher than the threshold requirement for juveniles to grow (0.02–0.10 μg C ml−1) (Ikeda and Thomas 1987) but certainly not as dense as has been described in some blooms (>8 μg Chla l−1; Tréguer and Jacques 1992). Since laboratory kept krill require a far higher food concentrations in the laboratory than has been observed in the natural condition to attain equivalent growth rate to the field (Ikeda and Thomas 1987), the food environment in our study was not at saturating level. In our study, krill kept in the large tank may expend more energy leaving less to be diverted to drive the maturation cycle resulting as their later maturity recovery compared to those kept in small jars.
Furthermore, while the female maturity stage was maintained at Stage-E once they re-matured under excess food conditions, starved krill started regressing as soon as they re-matured (Thomas and Ikeda 1987), or in some cases they even started regressing before they reached stage-E (this study). These results suggest that female krill need a certain amount of food at the time when they re-mature, otherwise they once again regress. This underscores the critical role of food availability, whether from sea-ice algae or the spring phytoplankton bloom, in the natural environment in the period leading up to the reproductive season.
A conceptual model for the seasonal krill maturity cycle
Euphausiids are obligate moulters and they are known to regularly moult for their entire lifespan. This suggests that the moulting event is an excellent unit for conceptualising krill life history. The advantage of a moulting-based lifetime model would be its capacity of incorporating environmental factors such as temperature trends and effect of food environment as we gather further information. For krill, there are several recent attempts to use this approach to conceptualise specific aspects of the krill life history (e.g. Cuzin-Roudy and Buchholz 1999; Candy and Kawaguchi 2006). There is of course still a long way to go to establish a comprehensive krill lifetime model, however, here we describe an initial conceptual model for seasonal krill maturity.
General progression of seasonal cycle of external sexual characteristics
The female external sexual characteristics, thelycum, undergoes a cycle starting from a fully developed spent female at the end of its reproductive season, regressing to an immature form, then re-maturing to a fully developed form regardless of food and light condition (Thomas and Ikeda 1987). This “regression cycle” is the period that krill need to restore and re-organise their ovary following a reproductive season (Makarov 1975; Cuzin-Roudy 2000). This makes ecological sense since it is unlikely that krill encounter feeding conditions to satisfy their reproductive needs during the winter period anyway.
The duration of the cycle (the total number of moulting events) may be affected by external factors such as food condition and temperature (Buchholz 1991; Kawaguchi et al. 2006). The pattern of the IMP within this cycle also varies, with relatively short IMPs at the start of regression, an increase as they regress, then they shorten again towards the end of this cycle (Thomas and Ikeda 1987; this study). The IMP is longer under lower temperature regime. Food limitation also prolongs IMPs (e.g. Buchholz 1991; Kawaguchi et al. 2006).
Factors affecting growth pattern
Increased temperature almost universally increases the growth rates of crustaceans without any evidence of an optimum temperature (Hartnoll 2001). However, optimal temperatures have been demonstrated for Thysanoessa longipes (Iguchi and Ikeda 2005) and Antarctic krill (Atkinson et al. 2006) and Euphausia pacifica shows a simple positive relation between growth and temperature (Iguchi and Ikeda 1995). Further accumulation of experimental and survey data with wider seasonal and geographical coverage are needed to correctly understand this problem in euphausiids.
The seasonal endogenous physiological cycle of krill also affects their growth. Antarctic krill females lower their growth rates during the reproductive season as they divert energy into reproduction (Kawaguchi et al. 2006). Females undergoing regression following the reproductive season have also been observed shrinking regardless of their feeding conditions in laboratory experiments (Thomas and Ikeda 1987).
Concept of ovarian cycle during the reproductive season
During the reproductive season, Northern krill are engaged in cyclical egg production tightly linked with their moult cycle, and Antarctic krill are also thought to have a similar progression. During the reproductive season, female krill recycles the ovary multiple times as long as the environment allows (Cuzin-Roudy and Buchholz 1999). There are two moult cycles within an ovarian cycle for northern krill. One of those is “spawning moult cycle” during which eggs are released during the pre-moult phase and the other is “vitellogenic moult cycle” during which yolk accumulation for the next egg batch is completed. These two cycles alternates one after another until the end of the spawning season (Cuzin-Roudy and Buchholz 1999). Although it is still unclear how many moult cycles are involved in a single ovarian cycle for Antarctic krill, it is likely to last more than one moult cycle with alternative vitellogenic moult cycles for lipid yolk accumulation (Cuzin-Roudy 2000). The important factors affecting the overall fecundity during a single season are not only food during the reproductive season but also energy accumulation in the period leading up to the reproductive season, i.e. spring (Siegel and Loeb 1995). Since krill exhaust most of lipid storage during winter (Hagen et al. 1996), the substantial amounts of energy required for gonad maturation and egg production are probably provided by ice biota during early spring and by phytoplankton blooms during late spring and throughout summer (Falk-Petersen et al. 2000). The ultimate size of the egg-batch is an exponential function of body size (Cuzin-Roudy 2000).
Antarctic krill begin previtellogenesis at the ice edge (Cuzin-Roudy and Labat 1992) which signals the start of the “reproductive season”, although whether the actual ice melt is the direct cue to initiate the ovarian cycle or some other indirect effects (e.g. increase of light penetration due to the clearance of ice, a decrease in salinity due to ice melt, the boost of food availability due to ice-edge bloom, or the cumulative food intake) are responsible is unknown. Neither are the cues that signal the end of the reproductive season known. However, since reproductive season has been demonstrated to extend up to 9 months under optimal laboratory conditions (Hirano et al. 2003), it would be reasonable to assume that the termination of the natural reproductive season is due to a shortage of energy accumulation to sustain the vitellogenic cycle.
The age structure of the spawning population is also suggested to influence the start of reproductive season since the second or third time spawners mate and mature earlier than the first time spawners (Cuzin-Roudy and Labat 1992; Spiridonov 1995). The readiness of the first time spawners are likely to be heavily dependent on the environmental conditions (food availability, temperature, etc.) leading up to the reproductive season.
The effect of light conditions remains uncertain, although if light is not a primary factor governing krill maturation (Hirano et al. 2003), then it certainly may have an indirect effect through regulating the seasonal pattern of primary production.
The seasonal conceptual model (Fig. 4)
Moulting events can be categorized into two types: one is the regression cycle during the resting (winter) season and the other is the ovarian cycles in the reproductive (summer) season. There are at least 10 moulting events during the regression cycle. The length of this period is governed by the magnitude of stage increments per moulting event, and the length and number of the intermoult periods, which are all primarily dictated by temperature and food conditions. After recovering its external maturity to stage-E, the females are ready to start the reproductive season and recycle their ovaries whenever the environment allows. The first time spawners reach this stage later than the older ones, and its timing depends on the environment. Here we assume ovarian cycles are alternate repetitions of a vitellogenic moult cycle and a spawning cycle. The model assumes that the reproductive season generally starts at the ice edge in spring. The duration of the reproductive season is dictated by the total energy intake (accumulation) from the spring until the diminishing of the food supply at the end of summer season (i.e. end of phytoplankton bloom). The availability of food is principally regulated by the natural cycle of the photoperiod, but is also affected by nutrient conditions, temperature trends, oceanography etc. The growth trajectory of krill can also be directly linked and related to the seasonal maturity cycle.
Conceptual model of krill maturity cycle in relation to environmental factors. R1, R2 number of moulting event since start of resting period; V vitellogenic moult cycle; S spawning moult cycle; Thick arrow general progression of female maturity; Thick dotted arrow maturity progression of a first time spawner; White circulating arrows ovarian cycle
As an example we describe the seasonal status of Scotia Sea krill using this model. Because of the relatively high temperature in this area and due to the abundance of sea-ice with ice algae as a food source during the winter, the overall IMP is short. During this preparatory period females are accumulating energy for reproduction through feeding on ice algae. Once the environment allows (the start of the ice retreat), the ovarian cycle starts.
Since the length of the summer is longer compared to higher latitudes, this gives a wide seasonal window available for reproduction and enables krill to sustain the ovarian cycle for prolonged period and as a consequence it contributes to overall high level of fecundity. Assuming krill follow temperature–growth relation generally known in crustaceans (Hartnoll 2001), then increased temperature may increase the growth rates. Also, larger females produce more eggs per female biomass (Cuzin-Roudy 2000). Thus, in the Scotia Sea where there is a long period of high phytoplankton abundance there would be a prolonged period of krill reproduction, higher growth rates resulting in larger females which in turn, because of their larger size, exhibit higher fecundity than female krill in the rest of their habitat which resides in higher latitudes. However at the same time, the known large inter-annual variability of sea-ice extension in the Scotia Sea underscores the highly variable nature of krill reproduction success in this area.
Conclusions
The model suggested through our current study emphasizes the importance of the winter environment which affects the duration of the regression cycle and the preparedness for reproduction. It further highlights the importance of the amount of sea ice algae available in the lead up to the reproductive season, and the timing of sea ice retreat in relation to the period when krill become physiologically ready to start their ovarian cycle. The overall length of the primary production season dictates the length of the period during which the ovarian cycles are sustained.
The model captures likely mechanisms of how the seasonality of the Antarctic environment may be linked to the endogenous physiological cycle of krill. We believe this conceptual model provides a useful framework to test various hypotheses regarding interactions between krill biology and the environment.
References
Atkinson A, Siegel V, Pakhomov E, Rothery P (2004) Long-term decline in krill stock and increase in salps within the Southern Ocean. Nature 432:100–103
Atkinson A, Shreeve RS, Hirst AG, Rothery P, Tarling GA, Pond DW, Korb RE, Murphy EJ, Watkins JL (2006) Natural growth rates in Antarctic krill (Euphausia superba): II predictive models based on food, temperature, body length, sex, and maturity stage. Limnol Oceanogr 51:973–987
Baker AdeC, Clark MR, Harris MJ (1973) The N.I.O combination net (RMT 1 + 8) and further development of Rectangular Midwater Trawls. J Mar Biol Ass UK 53:176–184
Bargmann HE (1945) The development and life-history of adolescent and adult krill Euphausia superba. Disc Rep 23:103–176
Buchholz F (1991) Moult cycle and growth of Antarctic krill Euphausia superba in the laboratory. Mar Ecol Prog Ser 69:217–229
Candy SG, Kawaguchi S (2006) Modelling growth of Antarctic krill II novel approach to describing the growth trajectory. Mar Ecol Prog Ser 306:17–30
Constable AJ, Nicol S, Strutton PG (2003) Southern Ocean productivity in relation to spatial and temporal variation in the physical environment. J Geophys Res 108 (C4). DOI 10.1029/2001JC001270
Cuzin-Roudy J (2000) Seasonal reproduction, multiple spawning, and fecundity in northern krill, Meganyctiphanes norvegica, and Antarctic krill, Euphausia superba. Can J Fish Aquat Sci 57(Suppl 3):6–15
Cuzin-Roudy J, Labat JP (1992) Early summer distribution of Antarctic krill sexual development in the Scotia-Weddell region: a multivariate approach. Polar Biol 12:65–74
Cuzin-Roudy J, Buchholz F (1999) Ovarian development and spawning in relation to the moult cycle in Northern krill, Meganyctiphanes norvegica (Crustacea: Euphausiacea), along a climate gradient. Mar Biol 133:267–281
Denys CJ, Mcwhinnie MA (1982) Fecundity and ovarian cycles of the Antarctic krill Euphausia superba (Crustacea, Euphausiacea). Can J Zool 60:2414–2423
Falk-Petersen S, Hagen W, Kattner G, Clarke A, Sargent J (2000) Lipids, trophic relationships, and biodiversity in Arctic and Antarctic krill. Can J Fish Aquat Sci 57(Suppl 3):178–191
Hagen W, Van Vleet ES, Kattner G (1996) Seasonal lipid storage as overwintering strategy of Antarctic krill. Mar Ecol Prog Ser 134:85–89
Hartnoll RG (2001) Growth in crustacean-twenty years on. Hydrobiologia 449:111–122
Hirano Y, Matsuda T, Kawaguchi S (2003) Breeding Antarctic krill in captivity. Mar Fresh Behav Physiol 36:259–269
Iguchi N, Ikeda T (1995) Growth, metabolism and growth efficiency of a euphausiid, crustacean Euphausia pacifica in the southern Japan Sea, as influenced by temperature. J Plankton Res 17:1757–1769
Iguchi N, Ikeda T (2005) Effects of temperature on metabolism, growth and growth efficiency of Thysanoessa longipes (Crustacea:Euphausiacea) in the Japan Sea. J Plankton Res 27:1–10
Ikeda T (1985) Life history of Antarctic krill Euphausia superba: a new look from an experimental approach. Bull Mar Sci 37:599–608
Ikeda T, Thomas T (1987) Moulting interval and growth of juvenile Antarctic krill (Euphausia superba) fed different concentrations of the diatom Phaeodactylum tricornutum in the laboratory. Polar Biol 7:339–343
Jackowski E (2002) Distribution and size of Antarctic krill (Euphausia superba DANA) in Polish commercial catches taken in the Atlantic sector of the Southern Ocean from 1997 to 1999. CCAMLR Sci 9:83–105
Kawaguchi S, Candy SG, King R, Naganobu M, Nicol S (2006) Modelling growth of Antarctic krill I Growth trends with sex, length, season and region. Mar Ecol Prog Ser 306:1–15
King R, Nicol S, Cramp P, Swadling KM (2003) Krill maintenance and experimentation at the Australian Antarctic Division. Mar Fresh Behav Physiol 36:287–299
Mackintosh NA (1973) Distribution of post-larval krill in the Antarctic. Disc Rep 36:95–156
Makarov RR (1975) A study of the second maturation of euphausiid (Eucarida, Euphausiacea) females (in Russian). Zoologicheskiy Zhurnal 54:670–681
Makarov RR, Denys CJ (1981) Stages of sexual maturity of Euphausia superba Dana. BIOMASS Handbook 11:1–11
Marr JWS (1962) The natural history and geography of the Antarctic krill (Euphausia superba Dana). Disc Rep 32:33–464
Marschall HP (1988) The overwintering strategy of Antarctic krill under the pack-ice of the Weddell Sea. Polar Biol 9:129–135
Nelson K (1991) Scheduling of reproduction in relation to molting and growth in malacostracan crustaceans. In: Wenner A, Kuris A (eds) Crustacean egg production, vol 7. Balkema, Rotterdam, pp 77–133
Poleck TP, Denys CJ (1982) Effect of temperature on the molting, growth and maturation of the Antarctic krill Euphausia superba (Crustacea: Euphausiacea) under laboratory conditions. Mar Biol 70:255–265
Port of Nagoya Public Aquarium (2002) Things that Antarctic krill tells us (In Japanese) News Letter Sakanakana. Port of Nagoya Aquarium. 34:1–4
Reid K (2001) Growth of Antarctic krill Euphausia superba at South Georgia. Mar Bio 138:57–62
Ross RM, Quetin LB (2000) Reproduction in Euphausiacea. In: Everson I. (ed) Krill biology, ecology and fisheries. Blackwell, Cambridge. pp 150–181
Siegel V (2005) Distribution and population dynamics of Euphausia superba: summary of recent findings. Polar Biol 29:1–22
Siegel V, Loeb V (1995) Recruitment of Antarctic krill Euphausia superba and possible causes for its variability. Mar Ecol Prog Ser 123:45–56
Siegel V, Loeb V, Bergstrom B, Scholing S, Haraldsson M, Kitchener J, Vortkamp M (2004) Demography of Antarctic krill in the Lazarev Sea (Subarea 48.6) and the Elephant Island area (Subarea 48.1) in 2004. SC-CAMLR WG-EMM-04/23
Smetacek V, Nicol S (2005) Polar ocean ecosystem in a changing world. Nature 437:362–368
Spiridonov VA (1995) Spatial and temporal variability in reproductive timing of Antarctic krill (Euphausia superba Dana). Polar Biol 15:161–174
Taki K, Hayashi T, Naganobu M (2005) Characteristics of seasonal variation in diurnal vertical migration and aggregation of Antarctic krill (Euphauia superba) in the Scotia Sea, using Japanese fishery data. CCAMLR Sci 12:163–172
Thomas PG, Ikeda T (1987) Sexual regression, shrinkage, re-maturation and growth of spent female Euphausia superba in the laboratory. Mar Biol 95:357–363
Tréguer P, Jacques G (1992) Dynamics of nutrients and phytoplankton, and fluxes of carbon, nitrogen and silicon in the Antarctic Ocean. Polar Biol 12:149–162
Acknowledgments
We thank A Constable for his useful discussion on the conceptual model. We also thank V Siegel and J Watkins for constructive comments on the earlier version of the manuscript.
Author information
Authors and Affiliations
Corresponding author
Rights and permissions
About this article
Cite this article
Kawaguchi, S., Yoshida, T., Finley, L. et al. The krill maturity cycle: a conceptual model of the seasonal cycle in Antarctic krill. Polar Biol 30, 689–698 (2007). https://doi.org/10.1007/s00300-006-0226-2
Received:
Revised:
Accepted:
Published:
Issue Date:
DOI: https://doi.org/10.1007/s00300-006-0226-2