Abstract
Lactic acid is a high-in-demand chemical, which can be produced through fermentation of lignocellulosic feedstock. However, fermentation of complex substrate produces a mixture of products at efficiencies too low to justify a production process. We hypothesized that the background acetic acid concentration plays a critical role in lactic acid yield; therefore, its retention via selective extraction of lactic acid or its addition would improve overall lactic acid production and eliminate net production of acetic acid. To test this hypothesis, we added 10 g/L of acetate to fermentation broth to investigate its effect on products composition and concentration and bacterial community evolution using several substrate-inoculum combinations. With rumen fluid inoculum, lactate concentrations increased by 80 ± 12 % (cornstarch, p < 0.05) and 16.7 ± 0.4 % (extruded grass, p < 0.05) while with pure culture inoculum (Lactobacillus delbrueckii and genetically modified (GM) Escherichia coli), a 4 to 23 % increase was observed. Using rumen fluid inoculum, the bacterial community was enriched within 8 days to >69 % lactic acid bacteria (LAB), predominantly Lactobacillaceae. Higher acetate concentration promoted a more diverse LAB population, especially on non-inoculated bottles. In subsequent tests, acetate was added in a semi-continuous percolation system with grass as substrate. These tests confirmed our findings producing lactate at concentrations 26 ± 5 % (p < 0.05) higher than the control reactor over 20 days operation. Overall, our work shows that recirculating acetate has the potential to boost lactic acid production from waste biomass to levels more attractive for application.
Similar content being viewed by others
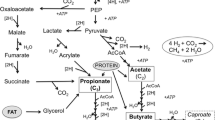
Explore related subjects
Discover the latest articles, news and stories from top researchers in related subjects.Avoid common mistakes on your manuscript.
Introduction
Lactic acid is a compound with versatile applications in many industries including food production, chemistry, textile, pharmaceutics and cosmetics. The global lactic acid market is forecasted to reach 367.3 kt by 2017, primarily due to the drive and demand from industry and new applications (GIA 2012). During the past decades, interest has grown in polylactic acid as a renewable and biodegradable plastic. However, due to the high cost of its precursor, lactic acid, use of this polymer has been limited. Lactic acid is currently produced at industrial scale through pure culture fermentation using filamentous fungi (e.g. Rhizopus spp.), bacteria (e.g. Bacillus coagulans) or yeast and using costly feedstock such as glucose (Taskila and Ojamo 2013a). Lactic acid accumulation acidifies the fermentation broth; therefore, much research has been carried out to genetically engineer microorganisms that are not only more tolerant to acids and low pH (below pH 4) but also utilize both pentose and hexose sugars, which are commonly found in lignocellulosic biomass (Taskila and Ojamo 2013b). Depending on the product application, mixed culture fermentation of lignocellulosic biomass can be an attractive alternative since it would eliminate the need for sterilization and utilize a cheaper substrate.
Lactic acid bacteria (LAB) usually ferment glucose into lactic acid. During homolactic fermentation, two molecules of lactate are produced per molecule of glucose via the formation of two molecules of pyruvate. During heterolactic fermentation, which typically occurs under substrate limitation, one molecule of lactate is produced via pyruvate, while one molecule each of ethanol and carbon dioxide are produced via acetyl-CoA. At either low pH or high substrate concentration, bacteria will undergo the shorter pathway of homolactic fermentation, to decrease demand for reducing power (Thomas et al. 1979). Many LAB are also able to degrade lactic acid to acetate under anoxic conditions in the presence of alternative electron acceptors or even under strict anaerobic conditions without supporting cell growth (Oude Elferink et al. 2001). When oxygen is present, pyruvate may be converted directly to acetate to benefit the cell (Quatravaux et al. 2006). Temudo et al. (2007) investigated open mixed culture fermentation of glucose under different pH to direct specific product formation since normally, a mixture of formate, acetate, butyrate and ethanol is produced. Acetate is typically the main side-product because production of acetate is energetically more favourable than lactate production (Hunt et al. 2010). Typically acetate concentrations vary widely in mixed culture fermentation (Elsden 1945; Wang et al. 2012). To completely eliminate acetate production, research has been done to genetically modified microorganisms such as Escherichia coli; however, small amounts are still detected even when the gene for acetate production is knocked out (De Mey et al. 2007). Bobillo and Marshall (1992) found that addition of salt (6 % NaCl) could inhibit acetate production in Lactobacillus plantarum at pH 4.5, without inhibiting lactate production. It has also been demonstrated that acetate can inhibit bacterial growth when present at high concentrations (>5 g/L) and low pH (<7) (Luli and Strohl 1990; Roe et al. 2002; Russell 1992), depending on the bacteria strain and operating conditions. If high but not toxic concentrations of acetate are present in open culture, it is possible that acetate accumulation will direct the biochemical processes towards other reactions. This may be a possible strategy to steer the bacterial community towards net lactate production. To enable this, a process which is able to extract and separate lactic acid and acetic acid is necessary.
Regarding the separation of lactic acid from acetic acid, numerous separation technologies have been investigated including electrodialysis, ion exchange and extractive distillation. Separation of lactic acid from acetic acid has also been demonstrated using a four-zone simulated moving bed process (Lee et al. 2004). Recently, a membrane electrolysis approach was developed that provides both specific extraction of unbranched fatty acids and pH control of the fermentation broth without chemical dosing (Andersen et al. 2014). This approach might be used to boost lactate production by separating acetate and lactate downstream of the fermentation and returning the acetate.
The objective of the current study was thus to investigate the effect of high acetate concentration on the fermentation products (both batch and semi-continuous mode) and bacterial community (batch mode) with the aim of driving the fermentation to lactic acid. A target of 10 g/L acetate was chosen as a realistic value to reach considering typical production values in mixed cultures (2.5 g/L acetate in the study of Wang et al. (2012)) and an extraction system where lactate is removed and acetate is recycled back to the reactor. To compare the performance between different substrates and inocula, fermentation of both a simple (cornstarch) and complex (extruded grass) substrate were tested using three different inocula: a pure culture of Lactobacillus delbrueckii, a genetically modified (GM) E. coli and a mixed microbial community from rumen fluid.
Materials and methods
Substrate
A readily fermentable substrate such as cornstarch (total solid (TS) 86.77 ± 0.05 %, volatile solid (VS, % of fresh mass) 86.35 ± 0.01 %, sold under the commercial name Basak) and a more lignocellulosic and complex substrate such as extruded grass (TS 64.14 ± 0.04 % and VS 60.35 ± 0.01 %) were used. Landscape grass was harvested on October 2012, kindly provided by Inagro vzw (West Flanders, Belgium). To make the grass more accessible for fermentation, it was extruded with a pilot scale twin-screw extruder (model MSZK, Laborextruder 4 kW, Lehmann, Germany), provided by Bioliquid (Raalte, the Netherlands), as performed by Khor et al. (2015). No further hydrolysis was performed. Substrates were stored at 4 °C until used.
Microorganisms and cultivation
L. delbrueckii LMG 6412 strain was obtained from the Belgian Co-ordinated Collections of Micro-organisms (BCCM) and grown in 1 L ‘de Man, Rogosa and Sharpe’ (MRS) medium at 37 °C. GM E. coli strain (3KO: E. coli K12 MG1655 δ (ackA-pta) δ (poxB), where the carbon flow to acetate is directly reduced), was provided by Marjan de Mey (InBio, Ghent University) and grown in 1 L Luria Bertani (LB) medium (De Mey et al. 2007) at 37 °C. Both strains were chosen as they have the ability to utilize both pure sugars and more complex substrates (Dumbrepatil et al. 2008; Rosales-Colunga and Martínez-Antonio 2014). Prior to inoculation, the cells were washed with M9 medium and concentrated to 50 mL through centrifugation (1500×g for 5 min) (De Weirdt 2013). Optical density (OD610) was 30.1 for L. delbrueckii and 10.2 for GM E. coli. M9 medium was composed of 8.5 g/L Na2HPO4, 3.0 g/L KH2PO4, 0.5 g/L NaCl, 1.0 g/L NH4Cl, 0.24 g/L MgSO4 and 0.011 g/L CaCl2. Rumen fluid (0.06 % bacterial protein) was provided by the Institute for Agricultural and Fisheries Research (ILVO), Ghent University. It was sieved and stored in a thermoflask before use on the same day.
Fermentation tests
Batch mode
Fermentations were carried out in 120 mL serum bottles for 8 days. In total, 20 different conditions and corresponding negative controls without substrate, each of them in triplicate, were performed, as shown in Table S1. For pure culture tests, each serum bottle contained 49 mL of M9 medium, 1 mL of concentrated bacteria (for L. delbrueckii and GM E. coli), 5 g of substrate (cornstarch or extruded grass) and 0.03 g of sodium bicarbonate to mitigate the stripping of CO2 into headspace. For mixed culture tests, each serum bottle was filled with 40 mL of M9 medium and 10 mL of rumen fluid. For tests with acetate addition, 683.5 mg sodium acetate (10 g/L acetate) was added. During the addition of substrate and inoculum, bottles were sparged with nitrogen to ensure anaerobic condition. The bottles were then flushed with nitrogen, except for a subset that was flushed with hydrogen. Gas samples were taken from the headspace of each bottle immediately after flushing to confirm that oxygen was removed. The pH was initially adjusted to either 5.5 for tests with rumen fluid and L. delbrueckii or 7 for tests with GM E. coli. The pH was not controlled throughout the experiment, but was measured at the end. As controls, auto-fermentation of extruded grass without inoculum addition was evaluated at pH 5.5 and pH 7, with and without acetate addition. All bottles were placed on a shaker (130 rpm) at 30 °C for 8 days. Gas production was monitored by means of pressure measurements, and liquid and gas samples were taken and analysed on days 0, 1, 2, 4 and 8 for each replicate. Samples for bacterial community analysis were taken on day 8.
Semi-continuous mode
Two vertical up-flow tubular acrylic glass reactors of 400 mL were packed with 30 g of extruded grass each and inoculated with 60 mL of rumen fluid. They were run under anaerobic condition for 50 days in a 20 °C room. M9 medium was circulated through the reactors at a flow rate of 0.1 mL/min. Half of the reactor outlet was recycled back to the reactor while the other half was purged as effluent (2 days of hydraulic retention time). For the test reactor, concentrated sodium acetate (100 g/L sodium acetate) was added in the inlet at a rate of 5 μL/min to replenish the loss of acetate in the effluent, achieving 10 g/L acetate in the reactor, while for the control reactor, there was no acetate addition. Half of the substrate was replaced with fresh substrate every 2 days, and the substrate was not mixed to ensure 4 days solid retention time. The reactors were sparged continuously with nitrogen during substrate replacement to ensure anaerobic environment. Sampling for chemical analysis was performed before the substrate was replaced (every 2 days), and average concentrations and standard deviations were calculated for all collected values.
Bacterial community analysis
DNA extraction
DNA extraction was performed using the FastPrep method described by Vilchez-Vargas et al. (2013). Samples were taken of inoculum, substrate and end of fermentation broth for analysis. Samples of 0.5 mL were centrifuged at 11,000×g for 5 min in a 2-mL Lysing Matrix E tube (Qbiogene, Alexis Biochemicals, Carlsbad, CA). Cell pellets were re-suspended in 1 mL of lysis buffer containing Tris/HCl (100 mM pH 8.0), 100 mM EDTA, 100 mM NaCl, 1 % (w/v) polyvinylpyrrolidone and 2 % (w/v) sodium dodecyl sulphate. Cells were lysed in a Fast Prep-96 homogenizer (40 s, 1600 rpm). Samples were then centrifuged at 18,000×g for 1 min at room temperature and washed once with one volume phenol/chloroform (1:1) and the second time with one volume chloroform. After centrifugation, nucleic acids (aqueous phase) were precipitated with one volume of ice-cold isopropanol and 1:10 volume of 3 M sodium acetate. After centrifugation and washing with 80 % ethanol, the pellet was re-suspended in 20 μL of Milli-Q water. The quality and quantity of the DNA samples were analysed on 1 % agarose gels.
DNA sequencing and bioinformatics processing
The V3–4 regions of the bacterial 16S rRNA gene was sequenced by Illumina sequencing MiSeq and v3 Reagent kit (http://www.illumina.com/products/miseq-reagent-kit-v3.ilmn, by LGC Genomics GmbH, Berlin, Germany) using 2 × 300 bp paired-end reads and primers 341F (5′-NNNNNNNNTCCTACGGGNGGCWGCAG) and 785R (5′-NNNNNNNNTGACTACHVGGGTATCTAAKCC) described in Stewardson et al. (2015). Each polymerase chain reaction (PCR) included approximately 5 ng of DNA extract and 15 pmol of each forward and reverse primer, in 20 μL volume of MyTaq buffer containing 1.5 units MyTaq DNA polymerase (Bioline) and 2 μL of BioStabII PCR Enhancer (Prokopenko et al. 2013). For each sample, the forward and reverse primers had the same 8-nt barcode sequence. PCRs included a pre-denaturation step of 2 min at 96 °C pre-denaturation step; followed by 30 cycles of the following: 96 °C for 15 s, 50 °C for 30 s and 72 °C for 60 s. DNA concentration of the amplicons of interest were determined by gel electrophoresis. About 20 ng of amplicon DNA from each sample were pooled for a total of 48 samples each carrying different barcodes. PCRs showing low yields were further amplified for 5 cycles. The amplicon pools were purified with one volume AMPure XP beads (Agencourt) to remove primer dimer and other small mispriming products, followed by an additional purification on MinElute columns (Qiagen). About 100 ng of each purified amplicon pool DNA was used to construct Illumina libraries using the Ovation Rapid DR Multiplex System 1-96 (NuGEN). Illumina libraries were pooled and size selected by preparative gel electrophoresis. Sequencing was done on an Illumina MiSeq using v3 Chemistry (Illumina).
Bioinformatics was conducted with 16S rRNA targeted metagenomics analysis (QIIME; (Caporaso et al. 2010)). Data were pre-processed by first de-multiplexing of all samples using Illumina’s CASAVA data analysis software version 1.8.4. The reads were then sorted by amplicon inline barcodes, no barcode mismatches were allowed. The barcode sequence was clipped from the sequence after sorting and reads with missing barcodes, and one-sided barcodes or conflicting barcode pairs were discarded. Sequencing adapters in all reads were removed and reads with final lengths below 100 nt were discarded (Clipping of Illumina TruSeq™ adapters in all reads). Combination of forward and reverse reads was done using BBMerge 34.30 (http://bbmap.sourceforge.net/). The sequence fragments were turned into forward-reverse primer orientation after removing the primer sequences. FastQC report was creating for every FASTQ files. 16S pre-processing and OTU picking from amplicons were performed using mothur v1.33 software package (Schloss et al. 2009). Sequences containing ambiguous bases (Ns), with homo-polymer stretches of more than eight bases or with an average Phred quality score below 33 were removed. Reads were aligned against the 16S Silva reference alignment release 102. Truncated and unspecific PCR products were removed. Fifteen thousand sequences per sample were subsampled. Pre-clustering allowed up to three differing bases in a cluster. Chimera was removed using uchime algorithm (Edgar et al. 2011). Taxonomical classification of sequences and removal of non-bacterial sequences were done using the Silva database. OTU were picked by clustering at the 97 % identity level using the cluster split method.
The raw sequence dataset was deposited on the European Nucleotide Archive (ENA) of European Bioinformatics Institute, with accession number LT006862–LT009376 (http://www.ebi.ac.uk/ena/data/view/LT006862-LT009376). Information regarding the OTUs are included in the supplementary material—OTU table (xls format).
Statistical analysis
Representation of principal coordinate analysis (PCoA) of Bray-Curtis dissimilarity indexes was performed. The Vegan package in R (R version 2.13.2, http://www.r-project.org/) was used to calculate Bray-Curtis dissimilarity matrices (vegdist function), and the data was represented by PCoA function using the ape package.
Analytical methods
Determination of fermentation products including lactate, acetate, propionate and butyrate was performed with Dionex ion chromatography equipped with IonPac ICE-AS1 column (Dionex) using 4 mM H2SO4 as eluent at a flow rate of 0.8 mL/min and an ED50 conductivity detector. The gas phase composition was analysed with a compact gas chromatography (Global Analyser Solutions, Breda, The Netherlands), equipped with a Molsieve 5A pre-column and Porabond column (for CH4, H2 and N2) and a Rt-Q-bond pre-column and column (for CO2). Nitrogen was used as carrier gas for H2 analysis while helium was used for CH4 and N2. Concentrations of gases were determined by means of a thermal conductivity detector. Bacterial protein of rumen fluid was analysed according to Makkar et al. (1982). Optical density (OD610) of pure culture inoculum was measured with a UV-vis spectrophotometer (ISIS 9000, Dr. Lange, Germany) at 610 nm.
Results
Impact of acetate addition on lactic acid concentration
Batch mode
Figure 1 depicts the results of fermentation tests with different inocula and substrates. When acetate was not added (w/o acetate), it was still detected as an intermediate at the end of the tests. However, in the test with acetate addition (w/ acetate), a smaller acetate production over the initially supplied was obtained (the difference between initial and end point was smaller than in w/o acetate tests) in most combinations. Using cornstarch, there was no statistical difference in terms of lactic acid production between tests with and without acetate addition when pure cultures were used as inoculum. However, with rumen fluid as inoculum, the final lactic acid concentration was 80 ± 12 % (p < 0.05) higher when acetate was added vs. control bottles. In experiments with extruded grass, acetate addition increased the lactic acid concentration after 8 days of fermentation by 16.7 ± 0.4 % (p < 0.05) with rumen fluid inoculum and by 4 to 23 % with either pure culture or genetically modified inoculum. There was no statistical difference in lactate production for non-inoculated extruded grass tests at pH 5.5 (p > 0.05) with or without acetate addition (Table 1). Although initially the pH was adjusted to 5.5, the final pH of all bottles supplemented with acetate reached an average of 4.4, while those without acetate addition reached 3.9, which roughly corresponds to the pK a of the respective major species present in solution (pK a acetic acid 4.75:pK a lactic acid 3.8).
The highest final lactate concentration (14.7 ± 0.3 g/L) was achieved with rumen fluid inoculum and extruded grass as substrate, coupled with 2.7 ± 0.1 g/L acetate production over that supplied (Fig. 1; Table S2). Elevated acetate concentrations did not alter the spectrums of other fermentation products such as butyrate and propionate (Figure S1). The initial rates of lactate production did not differ much with acetate addition (Figure S2). No methane was detected in all tests with nitrogen in the headspace.
Semi-continuous mode
A semi-continuous lactic acid fermentation of extruded grass gave results similar to those observed in batch mode, where supplemental acetate resulted in a higher final lactate concentration (Fig. 2). Reactors were started up allowed to acclimate during the first 30 days after which operation continued until day 50. On average over the latter fermentation period (20 days), the lactic acid concentration was 26 ± 5 % (p < 0.05) higher in the reactor with acetate addition, compared to that of the control reactor. The spectrums of fermentation products were similar to those observed in the batch fermentation tests, where they did not differ with or without the addition of acetate. Also, no methane was detected during the fermentation. Since the purpose of this test was to investigate the effect of elevated acetate concentration, process optimization was not performed, hence the low conversion and production rate. The average conversion achieved was 0.14 ± 0.01 g lactate/g VS extruded grass fed for the control reactor and 0.17 ± 0.01 g lactate/g VS extruded grass fed for the test reactor (p < 0.05). The average production rate was 2.0 ± 0.1 g/L/day for control reactor and 2.6 ± 0.2 g/L/day (p < 0.05) for test reactor. The operational conditions for the semi-continuous reactors were defined from the batch test outcomes. A solids retention time (grass) of 4 days was chosen since lactate concentration had reached a plateau after 4 days of fermentation in batch tests (Figure S2). Propionate and butyrate profiles are presented in Figure S3.
Effect of operational parameters on lactic acid production
Additional batch tests were carried out to evaluate the effect of operational conditions on lactate production, including headspace composition, pH and inoculum choice. First, hydrogen presence to create reductive conditions was tested. Cornstarch was used as substrate and rumen fluid as inoculum, with and without acetate addition, with either nitrogen or hydrogen in the headspace (Table 2). Hydrogen addition did not enhance lactate production but, even with a low working pH of 5.5, generated methane with and without acetate addition (3.18 ± 0.12 and 3.26 ± 0.36 mmol, respectively).
To evaluate pH and inocula, tests with extruded grass were performed with different inocula and their respective optimal pH (5.5 or 7). Controls without inoculum addition at pH 5.5 and 7 were also included to evaluate the performance of the autochthonous bacteria in the grass (Table 1). No differences in lactate (only about 4 g/L) were detected in the bottles without inoculum at different pH. However, when acetate was supplied, lactate only increased about 1 g/L at pH 5.5 (not statistically significant) while it improved by nearly 5 g/L at pH 7, which accounted for an increase of 111.1 ± 42.4 %. Thus, lactate titres were improved at higher pH, either with or without inocula. In most cases, inoculated bottles reached higher lactic acid concentration compared to the non-inoculated ones. When acetate was supplied, lactate production was again improved. Fermentation of extruded grass by inoculated with either rumen microbial community or L. delbrueckii maintained higher lactic acid concentrations than with GM E. coli. When looking at production rates (Figure S2), a 1-day lag time was observed before lactate concentration increased significantly in tests without inoculum, while lactate increased from day 0 in the inoculated bottles. Apart from the lag phase, the rate of production for both inoculated (3.9 ± 0.1 g/L/day, between day 0 and day 1) and non-inoculated bottles (4.1 ± 0.3 g/L/day, between day 1 and day 2) was similar.
Impact of acetate addition on bacterial community structure
Community shift with different substrates
The effect of elevated acetate on bacterial community of bottles inoculated with rumen fluid (both extruded grass and cornstarch) or without any inoculation (extruded grass only) was investigated and compared. A mixed microbial community such as ones found in rumen fluid can be modified from its initial structure by application of different operational conditions. Firstly, we measured the impact of acetate addition on fermentation of cornstarch and extruded grass as substrates (Fig. 3). Lactobacillus spp. became the most dominant species, with and without acetate addition for both substrates tested. Lactobacillus spp. is a lactic acid bacterium (LAB) able to convert sugars such as glucose and xylose, present in lignocellulosic biomass, to lactic acid. When no acetate was added, there were no significant differences in the microbial community between the tests with cornstarch and extruded grass (Fig. 3a versus Fig. 3c). When acetate was supplemented, the bacterial diversity increased, resulting in a decrease in the relative abundance of Lactobacillus spp. (e.g. 69 ± 3 %, Fig. 3b versus 84 ± 4 % in control bottles, Fig. 3a, p < 0.05). Also with acetate addition, a higher relative abundance of Prevotella spp. was found in tests with cornstarch (20 %) compared to extruded grass (2 %). Furthermore, when the two substrates both with acetate addition were compared, extruded grass gave more diverse lactic acid producers compared to cornstarch at the end of fermentation batch test (e.g. Fig. 3b, d), which likely correlates to higher feed complexity.
In rumen fluid inoculum itself, Prevotellaceae family was the most dominant (e.g. 85 % relative abundance, Figure S4a). Acidaminococcaceae (6 %) and Ruminococcaceae (2 %) were also present in lower abundance (Figure S4a). While on the extruded grass itself, a very diverse inherent bacterial community was found, with Curvibacter spp. (23 %), Massilia spp. (14 %) and Bacillus spp. (9 %) being the three most abundant species (Figure S4d). After 8 days of batch fermentation, most test and control bottles consisted mainly of LAB, especially Lactobacillus spp. (69 to 99 %), while its presence in both the rumen inoculum (0.03 %) and extruded grass (Figure S4d, below detection limit) were extremely low.
Effect of headspace composition on the microbiome
The bacterial community distribution in fermentations carried out on cornstarch as substrate and inoculated with rumen fluid was analysed under different headspace gas composition and acetate presence (Fig. 4). As we observed with different substrates, gas composition did not have any effect on the community structure and Lactobacillus spp. was by far the dominant species without acetate addition. Also in this case, regardless of the gas composition in the headspace, the addition of acetate increased the microbial diversity with the presence of 3–4 % Prevotella spp. (Fig. 4b, d). Primers used in these tests were specific to bacterial DNA; hence, methanogens were not detected in the sequencing.
Bacterial community under different pH and acetate presence
Chemical analysis already showed a lower lactic acid production in non-inoculated bottles compared to the inoculated during fermentation test with extruded grass. The bacterial community under fermentation at different pH and acetate concentrations was evaluated (Fig. 5). During fermentation at pH 5.5 with extruded grass and no inoculum, a more diverse bacterial community was obtained compared to samples inoculated with rumen fluid (e.g. Fig. 5a versus Fig. 3c). Initially on extruded grass, the Comamonadaceae family (26 %, Figure S4d) dominated the diverse mix bacterial community but over time, the fermentation resulted in a bacterial community mainly composed of LAB (52 ± 25 %, Fig. 5a). When acetate was elevated, LAB abundance increased further to 82 ± 29 % (Fig. 5b).
In batch experiments where extruded grass was fermented at pH 7 without inoculum, lower abundance of LAB was obtained, with Lactobacillus spp. making up 17 ± 15 % (Fig. 5c) and Leuconostoc spp. making up 4 ± 4 % (Fig. 5c). When acetate was supplemented, multiple strains of LAB dominated the bacterial community (89 ± 76 %, Fig. 5d). Apart from Lactobacillus spp., the other LAB detected were Leuconostoc spp., Lactococcus spp. and Pediococcus spp. By comparing the non-inoculated extruded grass fermentation, Leuconostoc spp. (LAB) represented a much higher proportion (34 ± 25 %, Fig. 5a) at pH 5.5 compared to at pH 7 (4 ± 4 %, Fig. 5c). Presence of Leuconostoc spp. was further stimulated by decrease of pH with the control bottles (Fig. 5b versus Fig. 5d), while the phenomenon was different for Lactobacillus spp., which was not perturbed by the pH (15 ± 3 % at pH 7, Fig. 5a versus 17 ± 15 % at pH 5.5, Fig. 5b). Data regarding alpha and beta diversity are presented in the supplementary material (Figures S5 and S6).
Discussion
Impact of acetate addition on lactic acid concentration
In most cases, elevated acetate concentrations decreased the net production of acetic acid. When different metabolic pathways are possible (i.e. lactate or acetate production), high product concentration will thermodynamically favour alternative pathways according to the Van’t Hoff equation (ΔG = ΔG° + RTlnQ). With regard to physicochemical properties, acetate addition will increase the conductivity of the system and high salt concentration can inhibit the growth of microorganisms (McCarty and McKinney 1961). Furthermore, acetate addition will bring the pH of the system towards its acidic buffer capacity (pK a 4.75), thus possibly favouring the route of the lactic acid production by LAB. However, a high proportion of the total acetate will be in equilibrium with the acid form of acetic acid, which may inhibit some microorganisms. By considering these effects, addition of acetate should have a positive impact on lactic acid fermentation if toxic concentrations are not reached. Takahashi et al. (1999) found that increasing acetate concentration (from 2 to 12 g/L) reduced the GM E. coli biomass concentration; however, the fermentation product (ethanol) concentration was not affected. In this study, addition of 10 g/L sodium acetate did not negatively affect the lactic acid production rate. On the contrary, initial rates seemed to be the same (Figure S2) and the addition of acetate increased the final lactic acid concentration. In the fermentation pathway, lactate production takes place via a relatively short and simple pathway to reduce pyruvate, generating one NAD+ for each pyruvate reduced. This pathway can quickly relieve the cell of reducing power by using lactate as electron sink, e.g. when substrate is over-abundant or during pH perturbation, although at the cost of lower energy gain (2.50–2.66 ATP per molecule glucose reduced) compared to acetate production. While producing acetate can yield more energy (4 ATP per molecule glucose reduced), lactate production can become the preferred pathway of microorganisms under these circumstances. Addition of acetate may perturb the reducing state of the environment, and hence stretch the lactate production further, as in this study.
Lactic acid can be degraded to acetate by many LAB, requiring electron acceptors and producing hydrogen (Quatravaux et al. 2006; Thauer et al. 1977). Thus, hydrogen presence should, by thermodynamically increasing the energy requirements of acetate production (Van’t Hoff equilibrium), assist in the shift from acetate to lactate production. However, no improvement was observed in the tests performed. While acetoclastic methanogenesis was inhibited during the fermentation tests under low pH (5.5), presence of carboxylates or short experimental time (8 days), low concentrations of methane were detected when hydrogen was supplied. Costa et al. (2013) studied the electron flow and energy conservation in hydrogenotrophic methanogens and showed that they are capable of using other substrates such as formate for growth, independently of hydrogen. Hence, this may result in substrate competition for lactate production and thus not notably increase its production.
Short hydraulic retention time (2 days) in combination with low pH (5.5) also allowed the suppression of methanogenesis. The tested reactor mimicked a continuous recirculation of acetate while lactate was harvested in the effluent. This is the first proof of concept that a selective extraction of lactate from acetate will enhance the titres and make the fermentation from (ligno)cellulosic biomass more feasible towards lactate production.
Effect of operational parameters: pH, inoculum and headspace gas composition
Fermentations inoculated with rumen culture or L. delbrueckii inoculum were set at pH 5.5 while those inoculated with GM E. coli were set at pH 7, based on the optimal working pH for the inoculum. This difference in pH could have an effect on the kinetics of the fermentation and caused the difference in lactic acid concentration (Fig. 1). In the test without inoculum, the end lactate concentration was similar without acetate addition at both pH tested. Lactic production was higher at pH 7 compared to pH 5.5 when acetate was added (Table 1), likely due to enhanced bacterial growth.
The pH only had a major impact when inoculum was not supplied. When the pH was low, more carboxylic acids (e.g. acetic acid and lactic acid) are in their protonated form. This allows the compounds to diffuse more easily into the bacteria cells, lowering the inner pH of bacterial cell. To combat this, bacteria have to invest more energy in cell maintenance and transporting the compounds out of the cell to maintain the inner cell pH. This caused energy loss which could otherwise be used to grow. Inoculation ensured that the fermentation was not inhibited due to low number of bacteria cells initially. Inoculation shortened the lag phase and allowed immediate conversion of readily digestible substrates into lactic acid (Figure S2). Comparing the three inoculums tested, rumen fluid inoculum had the best performance in terms of lactate concentration for both cornstarch and extruded grass as substrate. The mixed culture inoculum proved to be more effective for lactic acid fermentation on this complex substrate, probably due to a higher capacity to deal with the complex substrate.
Impact of acetate addition on bacterial community structure
Free acid inhibition of biochemical reactions is well documented (Colin et al. 2001). At high concentrations and low pH, carboxylate equilibrium shifts to the protonated form, which can penetrate the cell membrane and reduce the activity of most bacteria. Many studies have been performed on single strains of bacteria, such as Clostridium (Tang et al. 1989), and models have been developed to study the inhibitory effect of acetate (Zeng et al. 1994). However, the effect can be different for mixed microbial communities. In a study of a mixed community consisting of methanogens and acetogens, Fotidis et al. (2013) increased the acetate concentration stepwise up to 9 g/L and found that the growth of some cultures was inhibited, while that of others was either similar or significantly higher. In this study, a similar behaviour may occur as some bacteria only perform homolactic fermentation while many others can undergo heterolactic fermentation, and even lactate degradation, that will be affected by acetate concentration. Elevated acetate levels during fermentation of cornstarch promoted the presence of a more diverse bacterial community. A more specialized bacterial community, mainly composed of LAB, was found in the bottles without acetate addition (Table S3). However, the lactate concentration was higher in the bottles with acetate addition. This indicates that with acetate addition, more capacity for lactic acid production arose.
For fermentation of extruded grass without rumen fluid inoculum, an elevated amount of acetate promoted the presence of multiple species of LAB at pH 7, but not at pH 5.5 which may be due to the aforementioned higher relative proportion of its free acid form. Bobillo and Marshall (1992) studied the effect of acidic pH and salt on acid end products and found that L. plantarum is capable of altering its metabolic pathways of acid production as the environmental pH changes. Here, we observed rather a shift towards other bacteria maintaining the lactic acid fermentation. During fermentation of cornstarch, Lactobacillus spp. dominated the bacterial community and elevated acetate concentration gave rise to a more diverse bacterial community. Also, when rumen inoculum was used with extruded grass, the diversity of the bacterial community increased with elevated acetate concentration. Although the experiments performed here were likely too short to draw many conclusions on diversity in the longer term, the fact that similar chemical results were obtained for the semi-continuous operation could indicate that this likely remains the case in longer term. In the rumen fluid inoculum, Prevotellaceae family was the most dominant, followed by Acidaminococcaceae and Ruminococcaceae. These bacteria are commonly found among the species belonging to the rumen fluid core microbiome (Huws et al. 2015). Some Prevotella species have been reported to breakdown carbohydrates such as in Kabel et al. (2011), and they may have an important role in ruminal biohydrogenation (Huws et al. 2011).
Overall, it can be concluded that the addition of acetate to mixed culture fermentations geared at lactic acid production improves the rate and yield of the process while selecting for a community dominated by LAB. Although addition of acetate on mono-culture fermentation increased lactate production, the difference was not significant compared to bottles without acetate addition in some cases. The findings might be related to the capacity of mixed cultures to undergo different reactions and the thermodynamic impediment caused by acetate addition to heterolactic fermentation or lactic acid degradation.
References
Andersen SJ, Hennebel T, Gildemyn S, Coma M, Desloover J, Berton J, Tsukamoto J, Stevens C, Rabaey K (2014) Electrolytic membrane extraction enables production of fine chemicals from biorefinery sidestreams. Environ Sci Technol 48(12):7135–7142. doi:10.1021/es500483w
Bobillo M, Marshall VM (1992) Effect of acidic pH and salt on acid end-products by Lactobacillus plantarum in aerated, glucose-limited continuous culture. J Appl Bacteriol 73(1):67–70. doi:10.1111/j.1365-2672.1992.tb04971.x
Caporaso JG, Kuczynski J, Stombaugh J, Bittinger K, Bushman FD, Costello EK, Fierer N, Pena AG, Goodrich JK, Gordon JI, Huttley GA, Kelley ST, Knights D, Koenig JE, Ley RE, Lozupone CA, McDonald D, Muegge BD, Pirrung M, Reeder J, Sevinsky JR, Turnbaugh PJ, Walters WA, Widmann J, Yatsunenko T, Zaneveld J, Knight R (2010) QIIME allows analysis of high-throughput community sequencing data. Nat Methods 7(5):335–336. doi:10.1038/nmeth.f.303
Colin T, Bories A, Lavigne C, Moulin G (2001) Effects of acetate and butyrate during glycerol fermentation by Clostridium butyricum. Curr Microbiol 43(4):238–243. doi:10.1007/s002840010294
Costa KC, Lie TJ, Jacobs MA, Leigh JA (2013) H2-independent growth of the hydrogenotrophic methanogen Methanococcus maripaludis. Am Soc Microbiol 4(2):1–7. doi:10.1128/mBio.00062-13
De Mey M, Lequeux GJ, Beauprez JJ, Maertens J, Van Horen E, Soetaert WK, Vanrolleghem PA, Vandamme EJ (2007) Comparison of different strategies to reduce acetate formation in Escherichia coli. Biotechnol Prog 23(5):1053–1063. doi:10.1021/bp070170g
De Weirdt R (2013) Dietary fat and the human gut microbiome (PhD thesis). Ghent University, Faculty of Bioscience Engineering, Ghent, Belgium
Dumbrepatil A, Adsul M, Chaudhari S, Khire J, Gokhale D (2008) Utilization of molasses sugar for lactic acid production by Lactobacillus delbrueckii subsp. delbrueckii Mutant Uc-3 in batch fermentation. Appl Environ Microbiol 74(1):333–335. doi:10.1128/aem.01595-07
Edgar RC, Haas BJ, Clemente JC, Quince C, Knight R (2011) UCHIME improves sensitivity and speed of chimera detection. Bioinformatics 27(16):2194–2200. doi:10.1093/bioinformatics/btr381
Elsden SR (1945) The fermentation of carbohydrates in the rumen of the sheep. J Exp Biol 22:51–62
Fotidis IA, Karakashev D, Kotsopoulos TA, Martzopoulos GG, Angelidaki I (2013) Effect of ammonium and acetate on methanogenic pathway and methanogenic community composition. FEMS Microbiol Ecol 83(1):38–48. doi:10.1111/j.1574-6941.2012.01456.x
GIA (2012) Lactic acid—a global strategic business report http://www.strategyr.com/Lactic_Acid_Market_Report.asp. Accessed on 24 October 2014
Hunt KA, Flynn JM, Naranjo B, Shikhare ID, Gralnick JA (2010) Substrate-level phosphorylation is the primary source of energy conservation during anaerobic respiration of Shewanella oneidensis strain MR-1. J Bacteriol 192(13):3345–3351. doi:10.1128/JB.00090-10
Huws SA, Kim EJ, Lee MRF, Scott MB, Tweed JKS, Pinloche E, Wallace RJ, Scollan ND (2011) As yet uncultured bacteria phylogenetically classified as Prevotella, Lachnospiraceae incertae sedis and unclassified Bacteroidales, Clostridiales and Ruminococcaceae may play a predominant role in ruminal biohydrogenation. Environ Microbiol 13:1500–1512. doi:10.1111/j.1462-2920.2011.02452.x
Huws SA, Kim EJ, Cameron SJS, Girdwood SE, Davies L, Tweed J, Vallin H, Scollan ND (2015) Characterization of the rumen lipidome and microbiome of steers fed a diet supplemented with flax and Echium oil. Microb Biotechnol 8(2):331–341. doi:10.1111/1751-7915.12164
Kabel MA, Yeoman CJ, Han Y, Dodd D, Abbas CA, de Bont JAM, Morrison M, Cann IKO, Mackie RI (2011) Biochemical characterization and relative expression levels of multiple carbohydrate esterases of the xylanolytic rumen bacterium Prevotella ruminicola 23 grown on an ester-enriched substrate. Appl Environ Microbiol 77(16):5671–5681. doi:10.1128/aem.05321-11
Khor WC, Rabaey K, Vervaeren H (2015) Low temperature calcium hydroxide treatment enhances anaerobic methane production from (extruded) biomass. Bioresour Technol 176:181–188. doi:10.1016/j.biortech.2014.11.037
Lee HJ, Xie Y, Koo YM, Wang NH (2004) Separation of lactic acid from acetic acid using a four-zone SMB. Biotechnol Prog 20(1):179–192. doi:10.1021/bp025663u
Luli GW, Strohl WR (1990) Comparison of growth, acetate production, and acetate inhibition of Escherichia coli strains in batch and fed-batch fermentations. Appl Environ Microbiol 56(4):1004–1011
Makkar HPS, Sharma OP, Dawra RK, Negi SS (1982) Simple determination of microbial protein in rumen liquor. J Dairy Sci 65(11):2170–2173. doi:10.3168/jds.S0022-0302(82)82477-6
McCarty PL, McKinney RE (1961) Salt toxicity in anaerobic digestion. J Water Pollut Con F 33(4):399–415 Stable URL: http://www.jstor.org/stable/25034396
Oude Elferink SJWH, Krooneman J, Gottschal JC, Spoelstra SF, Faber F, Driehuis F (2001) Anaerobic conversion of lactic acid to acetic acid and 1,2-propanediol by Lactobacillus buchneri. Appl Environ Microbiol 67(1):125–132
Prokopenko MG, Hirst MB, De Brabandere L, Lawrence DJ, Berelson WM, Granger J, Chang BX, Dawson S, Crane EJ 3rd, Chong L, Thamdrup B, Townsend-Small A, Sigman DM (2013) Nitrogen losses in anoxic marine sediments driven by Thioploca-anammox bacterial consortia. Nature 500(7461):194–198. doi:10.1038/nature12365
Quatravaux S, Remize F, Bryckaert E, Colavizza D, Guzzo J (2006) Examination of Lactobacillus plantarum lactate metabolism side effects in relation to the modulation of aeration parameters. J Appl Microbiol 101(4):903–912. doi:10.1111/j.1365-2672.2006.02955.x
Roe AJ, O’Byrne C, McLaggan D, Booth IR (2002) Inhibition of Escherichia coli growth by acetic acid: a problem with methionine biosynthesis and homocysteine toxicity. Microbiology 148(7):2215–2222
Rosales-Colunga LM, Martínez-Antonio A (2014) Engineering Escherichia coli K12 MG1655 to use starch. Microb Cell Factories 13:74. doi:10.1186/1475-2859-13-74
Russell JB (1992) Another explanation for the toxicity of fermentation acids at low pH: anion accumulation versus uncoupling. J Appl Bacteriol 73(5):363–370. doi:10.1111/j.1365-2672.1992.tb04990.x
Schloss PD, Westcott SL, Ryabin T, Hall JR, Hartmann M, Hollister EB, Lesniewski RA, Oakley BB, Parks DH, Robinson CJ, Sahl JW, Stres B, Thallinger GG, Van Horn DJ, Weber CF (2009) Introducing mothur: open-source, platform-independent, community-supported software for describing and comparing microbial communities. Appl Environ Microbiol 75(23):7537–7541. doi:10.1128/aem.01541-09
Stewardson AJ, Gaïa N, François P, Malhotra-Kumar S, Delémont C, Martinez de Tejada B, Schrenzel J, Harbarth S, Lazarevic V (2015) Collateral damage from oral ciprofloxacin versus nitrofurantoin in outpatients with urinary tract infections: a culture-free analysis of gut microbiota. Clin Microbiol Infec 21(4):344.e1–344.e11. doi:10.1016/j.cmi.2014.11.016
Takahashi C, Takahashi D, Carvalhal M, Alterthum F (1999) Effects of acetate on the growth and fermentation performance of Escherichia coli KO11. Appl Biochem Biotech 81(3):193–203. doi:10.1385/ABAB:81:3:193
Tang IC, Okos MR, Yang S-T (1989) Effects of pH and acetic acid on homoacetic fermentation of lactate by Clostridium formicoaceticum. Biotechnol Bioeng 34(8):1063–1074. doi:10.1002/bit.260340807
Taskila S, Ojamo H (2013a) The current status and future expectations in industrial production of lactic acid by lactic acid bacteria
Taskila S, Ojamo H (2013b) The current status and future expectations in industrial production of lactic acid by lactic acid bacteria. R & D for food, health and livestock purposes, Dr. J. Marcelino Kongo (Ed.), ISBN: 978-953-51-0955-6, InTech, doi:10.5772/51282
Temudo MF, Kleerebezem R, van Loosdrecht M (2007) Influence of the pH on (open) mixed culture fermentation of glucose: a chemostat study. Biotechnol Bioeng 98(1):69–79. doi:10.1002/bit.21412
Thauer RK, Jungermann K, Decker K. (1977) Energy conservation in chemotrophic anaerobic bacteria. Bacteriol Rev 41(1):100–180 URL: http://www.ncbi.nlm.nih.gov/pmc/articles/PMC413997/
Thomas TD, Ellwood DC, Longyear VM (1979) Change from homo- to heterolactic fermentation by Streptococcus lactis resulting from glucose limitation in anaerobic chemostat cultures. J Bacteriol 138:109–117
Vilchez-Vargas R, Geffers R, Suarez-Diez M, Conte I, Waliczek A, Kaser VS, Kralova M, Junca H, Pieper DH (2013) Analysis of the microbial gene landscape and transcriptome for aromatic pollutants and alkane degradation using a novel internally calibrated microarray system. Environ Microbiol 15(4):1016–1039. doi:10.1111/j.1462-2920.2012.02752.x
Wang X, Li X, Zhao C, Hu P, Chen H, Liu Z, Liu G, Wang Z (2012) Correlation between composition of the bacterial community and concentration of volatile fatty acids in the rumen during the transition period and ketosis in dairy cows. Appl Environ Microbiol 78(7):2386–2392. doi:10.1128/aem.07545-11
Zeng AP, Ross A, Biebl H, Tag C, Günzel B, Deckwer WD (1994) Multiple product inhibition and growth modeling of Clostridium butyricum and Klebsiella pneumoniae in glycerol fermentation. Biotechnol Bioeng 44(8):902–911. doi:10.1002/bit.260440806
Acknowledgments
The authors would like to thank Charlotte Melis and Professor Veerle Fievez (ILVO, UGent) for providing the rumen fluid; Marjan de Mey (UGent) for providing the E. coli strain (3KO: E. coli K12 MG1655 δ (ackA-pta) δ (poxB); Lien Saey (InBio, UGent) for the analysis using liquid chromatography; Tim Lacoere for the figure illustration; Amanda Luther for proofreading of the manuscript; Sunil Patil, Emma Hernandez Sanabria and Chiara Ilgrande (LabMET, UGent) for their support; the Department of Bioengineering, Ghent University and the Department of Industrial Biological Science, Ghent University Campus Kortrijk.
Author information
Authors and Affiliations
Corresponding author
Ethics declarations
This article does not contain any studies with human participants or animals performed by any of the authors.
Conflict of interest
The authors declare that they have no competing interests.
Funding information
This work was funded by Special Research Fund (BOF, project number: DEF13/AOF/010) of the University of Ghent (Belgium). The funder has no role in study design, data collection and interpretation or the decision to submit the work for publication. MC was supported by Ghent University Multidisciplinary Research Partnership (MRP) – Biotechnology for a sustainable economy (01 MRA 510W). KR and HR are supported by the European Research Council Starter Grant ELECTROTALK.
Rights and permissions
About this article
Cite this article
Khor, W.C., Roume, H., Coma, M. et al. Acetate accumulation enhances mixed culture fermentation of biomass to lactic acid. Appl Microbiol Biotechnol 100, 8337–8348 (2016). https://doi.org/10.1007/s00253-016-7578-5
Received:
Revised:
Accepted:
Published:
Issue Date:
DOI: https://doi.org/10.1007/s00253-016-7578-5