Abstract
Ocean acidification, rising temperatures, and increased intensity of rain events are occurring due to climate change. Individually, each of these stressors has the potential to influence the growth and survival of many marine organisms, particularly during early development. Together the interactive and multiple impacts of elevated pCO2, temperature, and salinity may be exacerbated by a lack of food. Life history traits are important in determining the response of organisms to climate change. Larvae that develop within a brood chamber, such as the flat oyster, Ostrea angasi, may be pre-exposed to living a higher CO2 environment. This study determined the pH of the fluid surrounding the gills of adult oysters where larvae are brooded and investigated the interactive effects of the multiple climate-related stressors: ocean acidification, warming, hyposalinity, and reduced food availability, on development of O. angasi larvae. The fluid surrounding the larvae was of pH 7.88 ± 0.04, lower than that of surrounding sea water, and was significantly reduced (to pH 7.46 ± 0.05) when oysters remained closed as occurs in nature during periods of stress caused by low salinity. Elevated pCO2 [853–1194 µatm (pHNBS 7.79)] resulted in larvae being 3 % smaller, but it had no effect on the timing of progression through developmental stages, percentage of abnormalities, or survival of larvae. Exposure to elevated pCO2 together with increased temperature (+4 °C) or reduced salinity (20) had a negative effect on the time to the eyed larval stage and with an increase in the percentage of abnormal larvae. Unexpectedly, larvae did not meet their higher metabolic requirements to survive under elevated pCO2 by eating more. In a sublethal effect of elevated pCO2, larval feeding was impaired. We found that O. angasi larva were relatively resilient to elevated pCO2, a trait that may be due to the acclimatisation of hypercapnic conditions in the brood cavity or because they are released from the brood cavity at an older, possibly less sensitive stage. This result contrasts with the larvae of broadcast spawning oysters which are extremely sensitive to elevated pCO2.
Similar content being viewed by others
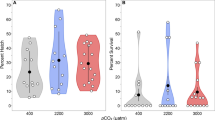
Explore related subjects
Discover the latest articles, news and stories from top researchers in related subjects.Avoid common mistakes on your manuscript.
Introduction
As a result of anthropogenic activities, oceanic conditions are changing placing stress on marine species and marine ecosystems (IPCC 2013, 2014). Increases in carbon dioxide levels in the atmosphere are exacerbating the multistressor world of marine organisms (Byrne and Przeslawski 2013). Increases in the atmospheric partial pressure of CO2 (pCO2) and its absorption by the ocean have resulted in a decrease in the saturation state of calcium carbonate and a decrease in the pH of the ocean (Feely et al. 2004; Orr et al. 2005; Doney et al. 2009; Mackenzie et al. 2014). This changing ocean chemistry, known as ocean acidification, is driving a decrease in calcification of marine species as a direct effect of the three co-varying stressors (pH, the saturation state of calcium carbonate, and pCO2), each which can impact biomineralisation (Byrne et al. 2013), interfering with the shell formation of calcifying molluscs (Gazeau et al. 2007; Wood et al. 2008; Thomsen et al. 2010). Independently, the three stressors associated with ocean acidification (pH, pCO2, and saturation state) can impact different aspects of the physiological processes of molluscs (Waldbusser et al. 2015a, b).
Ocean acidification has been shown to have mainly negative effects on a wide range of marine biota with calcifying species being negatively impacted, and molluscs ranking amongst the most vulnerable (Ries et al. 2009; Kroeker et al. 2010, 2013; Ross et al. 2011; Gazeau et al. 2013; Parker et al. 2013). The negative impacts of ocean acidification include decreased growth and calcification of adults (Ries et al. 2009), and reduced development and survival of larvae, including scallops (White et al. 2013; Scanes et al. 2014), clams (Talmage and Gobler 2011; Van Colen et al. 2012; Jansson et al. 2013; Gobler et al. 2014), mussels (His et al. 1989; Kurihara et al. 2009), and oysters (Parker et al. 2012; Barros et al. 2013).
Due to climate change, marine biota are also experiencing increased sea surface and aerial temperatures. Increasing atmospheric CO2 is predicted to cause a global increase in temperature of up to 4 °C by the 2100 (IPCC 2013). The effects of climate warming can exceed those of ocean acidification (Byrne et al. 2009; Talmage and Gobler 2011; Nguyen and Byrne 2014). Environmental temperature is a key determinant of the distribution of marine species due to the physiology of organisms operating within defined thermal windows (Helmuth and Hofmann 2001; Pörtner Pörtner 2008). Typically, growth and rates of development increase with warming (Zimmerman and Pechenik 1991; Byrne et al. 2009), and synergistic interactions are more evident with acidification (Parker et al. 2010; Ross et al. 2011; Hiebenthal et al. 2013; Matoo et al. 2013) than additive or antagonistic interactions with a broader suite of anthropogenic stressors (Przeslawski et al. 2015). For example, elevated pCO2 has been shown to reduce the growth of the blue mussel Mytilus galloprovincialis, but under moderate warming the effect was minimised (Kroeker et al. 2014) as also found for other calcifiers (Sheppard-Brennard et al. 2010). Furthermore, studies on metamorphosis of the Pacific oyster, Crassostrea gigas, found elevated temperature removed any negative effects of elevated pCO2 (Ko et al. 2014).
Broadscale climatic change will increase the frequency of storm events and subsequent coastal run-off and flooding in the catchment (IPCC 2013). Changes to salinity and coastal run-off due to rainfall will exacerbate other anthropogenic stressors such as nutrients, sedimentation, and metal contamination (Przeslawski et al. 2015), which are known to have negative impacts on marine invertebrates (Pedersen and Perkins 1986; Schone et al. 2003). Studies have also shown interactive effects of salinity with pCO2 on the development and physiology of oysters, but the results have varied. For example, for juvenile oysters, Crassostrea virginica, combined exposure to elevated pCO2 and reduced salinity weakened the shells and increased the consumption of energy (Dickinson et al. 2012). Furthermore, Ko et al. (2014) found interactive effects of decreased pH, elevated temperature, and reduced salinity slowed growth of the Pacific oyster, C. gigas. Conversely, Waldbusser et al. (2011) found that although calcification declined with elevated pCO2 in juvenile C. virginica, these negative effects were partially ameliorated by elevated salinity and temperature. For bivalves, the combined negative effects of increased temperature and decreased salinity on development, growth, and mortality are long recognised (e.g. Kinne 1971; Robert et al. 1988; His et al. 1989).
Increased food availability has the potential to ameliorate the energetic stress experienced by marine organisms due to acidification (Hettinger et al. 2013). Under stress, bivalve species have higher metabolic requirements to maintain homeostasis (Pörtner and Farrell 2008; Melzner et al. 2009, 2011). Increased food supply is reported to reduce the negative impacts of increased pCO2 on juvenile scallops and mussels (Sanders et al. 2013; Thomsen et al. 2013), and oyster larvae (Hettinger et al. 2013). Hettinger et al. (2013) found that the combination of elevated pCO2 and low food availability led to a large reduction in larval performance of Ostrea lurida. Thomsen et al. (2013) found that the Mytilus edulis was able to tolerate high (>3000 µatm) pCO2 when food supply was abundant. Thus, it appears that abundant food resources may partially ameliorate the negative impacts of ocean acidification on bivalves (Hettinger et al. 2013).
To be able to make predictions about which molluscs species will be most vulnerable in a future climate-changed ocean, it is essential to understand the additive, synergistic, or antagonistic effect of the multiple stressors associated with climate change (Byrne 2011). In a recent meta-analysis of multiple stressors on marine larvae and embryos, it was found that the majority were synergistic interactions (65 %), and that responses were taxon-specific (Przeslawski et al. 2015). Within bivalves, additive effects of pCO2 and temperature impact growth in the clam Mercenaria mercenaria and the oyster C. virginica (Talmage and Gobler 2011). Specifically, increases in temperature and pCO2 had negative and additive effects on the survival, development, and growth of larvae of these species (Talmage and Gobler 2011). For the oysters, Saccostrea glomerata and C. gigas, there were synergistic negative effects of pCO2 and temperature (Parker et al. 2009; Dickinson et al. 2012; Ko et al. 2014). Under the combination of elevated pCO2 and temperature, Parker et al. (2010) found reductions in size and development and an increase in the number of abnormally shaped larvae for both species of oysters.
For many marine molluscs with biphasic life histories, the early life stages have been shown to be the stage that is the most sensitive (Parker et al. 2010, 2011), which has led to concerns of early-stage bottlenecks and issues of persistence of populations (Byrne 2011). Some molluscs may, however, be relatively robust to ocean acidification due to pre-exposure to elevated pCO2 conditions. Parker et al. (2012) found that pre-exposure of adult oysters to ocean acidification during reproductive conditioning had positive “carry-over” effects and increased the resilience of their offspring. These carry-over effects persisted into the second generation, depending on their environmental exposure to pCO2 (Parker et al. 2015). Thomsen et al. (2010) also found that calcifying benthic species, including the mussel M. edulis, thrived in naturally CO2-enriched waters of the Western Baltic Sea. When placed under artificially elevated pCO2 in the laboratory, this long-term exposure to naturally elevated pCO2 increased the ability of M. edulis to calcify (Thomsen et al. 2010).
Some invertebrate species such as seastars that live in variable environments in tide pools are physiologically acclimated to withstand elevated pCO2 (Nguyen and Byrne 2014). The embryos and larvae of molluscs that rear their young within a brood chamber experience respiration-driven hypercapnic conditions during periods that the chamber is closed (Chaparro et al. 2008, 2009; Noisette et al. 2014). During stressful conditions, e.g. low salinity, females close off their brood chambers to protect the larvae, and the pH of the brood chamber can decrease to below pH 6.5 (Chaparro et al. 2009). Thus, the larvae of brooding bivalves maybe more tolerant of elevated pCO2 than larvae of broadcast spawning bivalves which are not naturally exposure to periods of hypercapnia. The larvae of the flat oyster, Ostrea angasi, are not within a brood chamber but initiate development on the gill filaments of the female and are likely to experience hypercapnic conditions as the respiratory pCO2 of water in the mantle cavity increases when the oyster is closed due to environmental conditions (Dix 1976). Larvae of species adapted to living in a brood, such as O. angasi, may have the phenotypic plastic characteristics that increase their acclimation capacity to respond to a high CO2 environment. The conditions experienced by larvae while brooded may therefore provide preconditioning to ocean acidification conditions. Gradual acclimation to elevated pCO2 has shown to allow preconditioning and provide less physiological and behavioural stress (e.g. intertidal isopods, Paradella dianae, Munguia and Alenius 2013). Thus, the progeny of brooding oysters may be more resilient to ocean acidification.
This study investigated the effect of multiple stressors: elevated pCO2, elevated temperature, hyposalinity, and reduced food availability on the larvae of the flat oyster, O. angasi. Flat oysters are a brooding species which retain developing larvae directly on the gill filaments and then release their young as planktotrophic larvae (Dix 1976; O’Sullivan 1978). In Australia and elsewhere, flat oysters are important aquaculture species (O’Connor and Dove 2009). Ostrea angasi occurs subtidally in coastal areas of relatively stable seawater salinities (Thomson 1954; Nell and Gibbs 1986), although heavy rainfall in south-eastern Australia during the summer spawning season often results in acute episodes of reduced salinity (hours to days). We predicted that there would be a reduction in the pH of the branchial area where the larvae are reared, during closure of adult shells. In ocean acidification experiments, we tested the hypothesis that the negative effects of elevated pCO2 on larval development, as widely reported for broadcast spawning molluscs, would be mitigated, in O. angasi due to the acclimation of brooded larvae to low pH conditions. In combination with warming, hyposalinity, and reduced food availability, it was predicted that there would be synergistic effects on the size, progression of development to the eyed larval stage, survival, and in the percentage of abnormally developed larvae. Additionally, due to the higher metabolic requirements of responding to stress, it was hypothesised that acidification, warming, and hyposalinity would increase food clearance rates by O. angasi larvae.
Methods
Determination of the pH of the pallial cavity of adult oysters
Adult O. angasi of the same age (3 years) were collected from farmed oyster leases at Port Stephens (32°42′38′′S, 152°4′5′′E) on the mid-coast of New South Wales. The oysters were maintained in the Port Stephens Fisheries Institute. To determine the pH of the pallial cavity, adult oysters were either forced to remain closed, simulating a stressful event such as hyposalinity, or left unmanipulated (e.g. Chaparro et al. 2009). The acute exposure reflects what occurs in nature when the salinity changes rapidly with heavy rain during February where these oysters are grown (Roy et al. 2001). Individual oysters were placed in 10-L buckets with 3 L of 1-µm filtered sea water (salinity 35.5; pHNBS 8.16 ± 0.02). They were left for 20 min until they were no longer disturbed and visibly open. Water was gently pumped into each bucket, so the oysters were not disturbed, with either sea water or freshwater to a volume of 7 L. For buckets of low salinity, salinity was dropped to 20 as monitored by a WTW 3420 metre with SenTix 940 probe.
The larvae of Ostrea spp. are free to move around inside the pallial cavity and are closely associated with the gills of their mothers (Mardones-Toledo et al. 2015). The pH of the fluid surrounding the gills where the larvae are held was measured by carefully drilling a 1-mm hole into the shell (Crenshaw 1972) and carefully extracting the fluid with a needle. The needle was inserted through the hole, avoiding the main body of the oyster, to the approximate mid-point between the inner surfaces of the two valves, to minimise the risk of the collection of either haemolymph or extrapallial fluid. As the larvae of O. angasi are directly brooded on the gills, the fluid that was sampled is that of which is surrounding the larvae whist within the adult. Approximately 0.5 mL of the fluid was extracted from each oyster and placed into a 2-mL microcentrifuge tube, ensuring a minimum of 5 mm of the pH microprobe was submerged in the fluid. The pH of the fluid was determined using a pH metre and microprobe (Metrohm 826 pH mobile with Metrohm 6.0224.100 microprobe). This was done for five oysters from each of the treatments immediately (<5 min) after the salinity had dropped (time 0), and after 1 h (time 1). During this time, oysters were observed to ensure they either remained open in the ambient sea water or closed in the reduced salinity treatment.
Untransformed data were analysed with a two-factor analysis of variance (ANOVA) in GMAV 5 (Underwood and Chapman 2002), with time (two levels) and salinity (two levels) as fixed and orthogonal factors, and n = 5 replicate oysters. Prior to analysis, the data were tested for homogeneity of variances, Cochran’s test. Based on the hypothesis of interest, Student–Newman–Keuls (SNK) tests were used to determine the direction of difference relative to the interaction of the factors of time and salinity.
Interactive effects of pCO2, temperature, salinity, and food on larvae
Collection of larvae
Female O. angasi (n = 27) obtained from a farm in from Wagonga Inlet (36°13′18′′S, 150°7′15′′E) were relaxed with MgCl (50 g L−1) in 25 % sea water with 75 % freshwater and then rinsed with 1-µm filtered sea water, and the larvae were gently rinsed from the brachial chamber (Butt et al. 2008). Prior to the experiments, all larvae were maintained in Port Stephens Fisheries Institute hatchery using standard larval rearing protocols (O’Connor et al. 2012). Late-stage larvae (shell length 257 ± 2 µm) were selected for the experiment to maximise the amount of time the larvae were within the adults and were at the umbonate stage that are released into the water column and start feeding (Dix 1976; O’Sullivan 1978). Larvae of this stage have developed on the gill filaments for 15–18 days (O’Sullivan 1978; Hickman and O’Meley 1988), and metamorphose 10–13 days after release (O’Connor et al. 2014). These larvae were used in either the multistressor experiment or the experiment to determine clearance rates.
Experimental design
To determine the effects of multiple stressors on the larvae of O. angasi, the four factors pCO2, temperature, salinity, and food were tested (Table 1). Each treatment had two levels: ambient pCO2 (388–417 µatm, equivalent to pHNBS 8.2, Table 2) or elevated pCO2 (1158–1206 µatm, equivalent to pHNBS 7.79, Table 2), optimum (26 °C) or elevated temperature (30 °C), ambient (32) or low salinity (20) (Table 2), and full (50,000 cells mL−1) or half diet (25,000 cells mL−1). The elevated pCO2 level was based on predictions under a medium-level scenario corresponding to the mean global surface pH predicted under scenario RCP8.5 for 2100 (IPCC 2013, 2014). The levels for each treatment of salinity, temperature, and food were based on the previous studies (O’Connor et al. 2012, 2014). Temperatures were based on the optimum temperature for rearing larvae of O. angasi under hatchery conditions (O’Connor et al. 2014), or a 4 °C increase in temperature under a high scenario for 2100 (IPCC 2014). The salinity was either ambient or reduced to a level experienced in the estuaries where O. angasi are grown and previously shown to decrease growth (O’Connor et al. 2014). The larvae were either fed a full food diet for maximum growth under hatchery conditions or a diet of half that amount of food known to results in decreased growth within the sublethal range for the larvae of O. angasi (O’Connor et al. 2012).
Sea water was trucked in from the Port Stephens coast and filtered to 1 µm. The elevated pCO2 level was obtained through adjustment of the seawater pH by direct bubbling of pure carbon dioxide (CO2) into sea water ( et al. 2012). The total alkalinity (TA) was quantified in triplicate by Gran titration prior to each water change (mean TA = 2285 ± 36 μmol kg−1; Gran 1952; Butler 1982). Salinity, temperature, and pHNBS were measured with each daily water change and remained constant throughout the experiment (Table 2). Following the titrations, the TA, salinity, temperature, and pHNBS were entered into a CO2 system calculation program (CO2 SYS, Lewis and Wallace 1998), pCO2 using the dissociation constants of Mehrbach et al. (1973).
The filtered sea water had a salinity of 32 throughout the study and is referred to hereafter as ambient salinity. The salinity of the water was decreased from ambient to 20 by adding deionised freshwater and then monitoring salinity changes with a WTW 3420 meter with SenTix 940 probe. Larvae were not acclimated to salinity over time to reflect the natural conditions that they would experience (Roy et al. 2001). The change in pH with decreasing salinity was also measured with a WTW 3420 metre with SenTix 940 probe (Table 2).
Larval rearing temperature was set to the optimal (26 °C) or elevated (30 °C) temperature (O’Connor et al. 2014) using thermostatically controlled water baths. Each 120-mL polypropylene container with larvae was randomly allocated to one of the three 500-L tanks set at 26 or 30 °C. With each daily water change, pH, salinity, and temperature were monitored to insure these experimental conditions remained consistent throughout the experiment (Table 2).
Larvae were fed either the full food diet (50,000 algal cells mL−1) or half food diet (25,000 algal cells mL−1) of the microalga, Isochrysis aff. galbana (T. Iso CS-177) daily. Microalgae used in the trial were cultured semi-continuously in 10-L polycarbonate vessels (Aquatek, SA), under cool-white fluorescent light exposed to a 16:8 h, light–dark photoperiod at 23 ± 1 °C using f/2 growth media (Guillard 1975). Microalgal cell concentrations in the algal cultures were determined every second day by triplicate cell counts using a Neubauer haemocytometer. Experiments were conducted in the dark to limit the potential impacts of algal photosynthesis on pCO2, but no differences in the pH of the water within each of the containers were detected after each 24-h period between water changes (Table 2).
At the start of the experiment, the mean size of larvae was determined from measurement of 30 randomly chosen individuals (shell length 257 ± 2 µm) using an eyepiece micrometer at 40× magnification under a compound light microscope. Larvae were stocked in tightly sealed 120-mL polypropylene containers at a concentration of 2 mL−1. After 4 days, 10 mL of 5 % formalin buffered with sea water was added to each container. The effects of temperature, pCO2, salinity, and diet on the final size, percentage of abnormalities (larvae exhibiting abnormal shell growth), percentage of larvae developing an eyespot, and larval mortality were determined from the assessment of 30 randomly encountered individuals per replicate container. There were n = 3 replicate containers for each combination of treatments.
Data analysis
The interactive effects of pCO2, temperature, salinity, and availability of food were tested. Data were analysed with a four-factor analysis of variance using GMAV 5 (Underwood and Chapman 2002). Each factor was orthogonal, fixed, and had two levels: ambient (297–394 µatm) or elevated pCO2 (853–1194 µatm), optimum (26 °C) or elevated temperature (30 °C), ambient (32) or low salinity (20), and full (50,000 cells mL−1) or half food diet (25,000 cells mL−1), n = 3 replicates of each treatment. Prior to analyses, the data were tested for homogeneity of variances with Cochran’s C test. To satisfy the assumption of homogeneity of variances, and to make comparisons among the different variables, the data for percentages of abnormalities, mortality, and eye larvae were ArcSin-transformed. Data that did not satisfy the assumption of homogeneity of variances were still analysed and interpreted with caution (α = 0.01), as large balanced ANOVAs such as these are robust to such violations (Underwood 1997). SNK tests were done for significant sources of variation to determine the patterns of difference relative to hypotheses of interest (Underwood 1997; Underwood and Chapman 2002).
Clearance rates of algae
The effects of pCO2, temperature, and salinity on clearance rates of food by larvae were determined over a 12-h period on larvae that were the same size as those used for the multistressor experiment. Experimental treatments comprised the orthogonal treatments of ambient or elevated pCO2, optimal or elevated temperature, and ambient or low salinity (Table 1). Following the previously described procedure, 120-mL polypropylene container was stocked with two larvae mL−1. Experimental controls consisted of the same treatments without the addition of larvae, also with n = 3 replicate containers per treatment. In each jar, 50,000 cells mL−1 of Isochrysis aff. galbana was added, and after 12 h, the experiment was stopped with the addition of formalin. The number of algal cells remaining in each container was determined, and larval clearance rates (cells larvae−1 h−1) were calculated (Coughlan 1969).
For the clearance rate experiment, untransformed data were analysed with a three-factor ANOVA (Underwood and Chapman 2002), testing for fixed and orthogonal treatments of pCO2, salinity, and temperature, n = 3 replicates of each treatment. Prior to the analysis, data were tested for homogeneity of variances. Post hoc SNK tests were done to determine the patterns of difference (Underwood 1997).
Results
Determination of the pH of the pallial cavity of adult oysters
At the beginning of the experiment, the mean ± SE pH of the fluid surrounding the gills of adult oysters was 7.88 ± 0.04, considerably lower than ambient sea water (pH 8.16 ± 0.02). The pH of the fluid surrounding the gills of adult O. angasi that remained opened did not change. For oysters that were closed for 1 h, the pH of the fluid surrounding the gills was lower, dropping to a mean ± SE pH of 7.46 ± 0.05 (n = 5; range 7.39–7.64 pH; Table 3).
Interactive effects of pCO2, temperature, salinity, and food on larvae
Effects on size
The effect of food on the size of larvae was contingent on pCO2 conditions (Table 4a). Larvae reared in ambient conditions were 3 % larger when fed the full diet (full food: 280 ± 4 μm, half food: 271 ± 2 μm, Fig. 1; Table 4a). Under elevated pCO2 conditions, larvae fed a full or half food diet were similar in size (Fig. 2; Table 4a). Although larvae under elevated pCO2 and a full food diet were significantly smaller than under ambient conditions, there was only a 3 % reduction in size (Fig. 2). Although larvae under elevated pCO2 and a full food diet were significantly smaller than under ambient conditions, there was only a 3 % reduction in size.
Mean (+SE, n = 3) shell length (µm) of larvae under a ambient pCO2 (390 µatm), or b elevated pCO2 (856 µatm), from optimum (26 °C) or elevated (30 °C) temperature, and ambient (32) or reduced (20) salinity, and fed either the full diet (50,000 cells mL−1; black bars) or half diet (25,000 cells mL−1; grey bars). Analyses were based on the mean shell length of 30 randomly chosen individuals from each replicate container. Letters represent significant differences (SNK P < 0.05) for the significant interaction between pCO2 and food (ANOVA P < 0.05, Table 4a)
Mean (+SE, n = 3) percentage of larvae that had reached the eyed stage of development jar−1 under a ambient pCO2 (390 µatm), or b elevated pCO2 (856 µatm), from optimum (26 °C) or elevated (30 °C) temperature, and ambient (32) or reduced (20) salinity, and fed either the full diet (50,000 cells mL−1; black bars) or half diet (25,000 cells mL−1; grey bars). Analyses were based on the percentage of 30 randomly chosen individuals from each replicate container. Letters represent significant differences (SNK P < 0.05) for the significant interaction amongst all factors (ANOVA P < 0.05, Table 4b)
Salinity and temperature interacted with each other to influence the size of larvae (Table 4a). For both the optimum and elevated temperatures, larvae were significantly smaller under reduced salinity conditions (Fig. 1; Table 4a). The difference between salinity treatments was greater under elevated temperature (Fig. 1). In ambient salinity, elevated temperature significantly increased the size of larvae (Fig. 1; Table 4a). The pattern was reversed for larvae in reduced salinity conditions such that larvae were significantly larger in the optimum temperature than in the elevated temperature (Fig. 1; Table 4a).
Effects on development
All four factors, pCO2, temperature, salinity, and food, interacted with each other to influence the percentage of larvae that reached the eyed stage of development (Table 4b). Specifically, there was no effect of pCO2 on development in the absence of other stressors, i.e. comparing treatments of ambient pCO2 with elevated pCO2 in combination with ambient salinity, optimum temperature, and full food diet (Fig. 2; Table 4b). Elevated pCO2 did, however, have a negative effect on development to the eyed larval stage when they were reared in ambient salinity and optimum temperature and were fed the half food diet (Fig. 2; Table 4b). Exposure to elevated pCO2 combined with hyposalinity (Fig. 2; Table 4b), or elevated temperature resulted in a decrease in the percentage of larvae that developed to the eyed larval stage.
Under the combined stress of reduced salinity and elevated temperature, the percentage of eyed larvae was also very low. There was no difference between ambient and elevated pCO2 conditions on the percentage of eyed larvae (Fig. 2; Table 4b). Furthermore, these differences were even greater when larvae were fed the half food diet (Fig. 2).
Effects on abnormalities
All four factors combined influenced the percentage of abnormally developed larvae (Table 4c). There was no effect of elevated pCO2 on the percentage of abnormal larvae when all other stressors were absent, i.e. ambient salinity, optimum temperature, and full food diet (Fig. 4; Table 4c). With the addition of any of the other stressors (reduced salinity, elevated temperature, or the half food diet), the percentage of abnormalities was greater under elevated than the ambient pCO2 (Fig. 3; Table 4c). This pattern was not statistically significant (Table 4c) for the treatment with reduced salinity, elevated temperature, and the full food diet, but the trend was in the same direction such that there was a greater percentage of abnormalities under elevated pCO2 (Fig. 3).
Mean (+SE, n = 3) percentage of abnormally developed larvae jar−1 under a ambient pCO2 (390 µatm), or b elevated pCO2 (856 µatm), from optimum (26 °C) or elevated (30 °C) temperature, and ambient (32) or reduced (20) salinity, and fed either the full diet (50,000 cells mL−1; black bars) or half diet (25,000 cells mL−1; grey bars). Analyses were based on the percentage of 30 randomly chosen individuals from each replicate container. Letters represent significant differences (SNK P < 0.05) for the significant interaction amongst all factors (ANOVA P < 0.01, Table 4c)
Effects on mortality
Hyposalinity and warming increased mortality of larvae, but this was not affected by the level of pCO2 or amount of food (Fig. 4; Table 4d). Overall, mortality was greater under the combined effects of reduced salinity and elevated temperature (Fig. 4). Furthermore, the effect of salinity on mortality of larvae was dependent on the temperature. Under the optimum temperature, there was no effect of salinity on mortality of larvae (Fig. 4; Table 4d). Under elevated temperature, mortality was significantly greater in the reduced salinity treatments (Fig. 4; Table 4d).
Mean (+SE, n = 3) percentage of larvae that died jar−1 under a ambient pCO2 (390 µatm), or b elevated pCO2 (856 µatm), from optimum (26 °C) or elevated (30 °C) temperature, and ambient (32) or reduced (20) salinity, and fed either the full diet (50,000 cells mL−1; black bars) or half diet (25,000 cells mL−1; grey bars). Analyses were based on the percentage of 30 randomly chosen individuals from each replicate container. Letters represent significant differences (SNK P < 0.05) for the significant interaction between salinity and temperature (ANOVA P < 0.01, Table 4d)
The effect of temperature on mortality of larvae also depended on salinity. Under ambient salinity, temperature did not affect mortality of larvae (Fig. 4; Table 4d). Under reduced salinity, mortality was significantly greater in the elevated temperature treatments (Fig. 4; Table 4d).
Clearance rates of algae
Under elevated pCO2, larvae had reduced clearance rates (Fig. 5). Specifically, larvae cleared fewer algal cells within the 12-h period in the elevated pCO2 compared to the ambient pCO2 (Fig. 5; Table 5). Clearance rates were also reduced with elevated temperature (Fig. 5; Table 5) and reduced salinity (Fig. 5; Table 5).
Discussion
This study found that when adult O. angasi close, even for short periods of time, the reduced pH conditions experienced by their brooded larvae are well in excess of the conditions expected under future models of ocean acidification for 2100. The pH of the fluid within the pallial cavity that larvae encounter was much lower than that of the ambient sea water (7.88 pH units).
Overall, the negative impacts of elevated pCO2 (853–1194 µatm) as a single stressor were relatively minor, with no effects on the timing of development, abnormality, and mortality of O. angasi larvae. There was an effect of elevated pCO2 on the size of larvae, with larvae being 3 % smaller compared to those reared under ambient pCO2. Effects on larval size were exacerbated by additional stressors including reduced food (that was half the diet required for optimum hatchery conditions), reduced salinity (reduced down from 32 to 20 which is encountered by O. angasi during heavy rain), and elevated temperature (4 °C increase predicted for 2100). There was delayed development, reduced size, and an increase in the prevalence of abnormal larvae reared in combined stressor treatments. Regardless of the presence of other stressors, there was no effect of elevated pCO2 on mortality of larvae.
Larvae were significantly smaller when reared under elevated pCO2, exhibiting a 3 % reduction in final size. Under similar conditions, however, the impact of elevated pCO2 on the growth of broadcast spawning bivalves was much greater. For example, elevated pCO2 resulted in up to a 65 % decrease in growth of larvae of S. glomerata ( et al. 2011), and decreases of 18 and 8 % for the clams, Argopecten irradians and M. mercenaria, respectively (Gobler and Talmage 2013). In contrast to the robust response of the larvae of O. angasi to elevated pCO2, larvae of broadcast spawning bivalves reared in the same conditions used here are extremely sensitive to acidification (Kurihara et al. 2007; Kurihara 2008; et al. 2010; Talmage and Gobler 2011). Elevated pCO2 as a single stressor did not result in negative impacts on the timing of development of O. angasi, abnormality or on mortality of larvae. The robust nature of the larvae of O. angasi to elevated pCO2 may be due to their acclimation to episodes of hypercapnia or because they are released at an older, possibly less sensitive stage. We used these later-stage larvae which are typically more robust than early-stage larvae (Gibson et al. 2011). Parker et al. (2010) did, however, use this umbonate larval stage and noted a reduction in size and increase in abnormal morphology of larvae under elevated pCO2 at the levels used here in S. glomerata and C. gigas.
Under ambient conditions, the fluid surrounding the larvae of O. angasi attached to the gills remained lower than the surrounding sea water (7.88 compared with 8.20 pH units). While it may be possible sample contamination by small amounts of extrapallial fluid and haemolymph occurred when pallial fluid was extracted from drilled oysters, it may also be influenced by respiratory CO2. When the individuals were closed for at least an hour, as occurs in response to heavy rain where O. angasi is farmed, the pH larvae experienced was lower (to pH 7.46) than that expected under extreme scenarios of elevated pCO2 for 2100 (IPCC 2014). While closure of adults for long periods may result in hypercapnic conditions which are detrimental to the survival of the larvae (Chaparro et al. 2009; Noisette et al. 2014), acute episodic exposure of larvae to reduced pH prior to liberation from the pallial cavity may be beneficial to larvae as our oceans continue to decrease on pH over this century. Similar resilience of larvae to elevated pCO2 occurs for broadcast spawners following laboratory exposure of their parents to elevated CO2 prior to spawning. For example, larvae of the oyster, S. glomerata, exhibited lower impacts of elevated CO2 on their growth, development, and abnormality (but not survival) if their parents were also reared at elevated compared to ambient pCO2 (Parker et al. 2012). Similar results were found in larvae of the sea urchin, Strongylocentrotus droebachiensis, which displayed no reduction in survival if their parents were reared at elevated compared to ambient pCO2 for 16 months prior to spawning (Dupont et al. 2013). It is unknown whether similar exposure of O. angasi parents to elevated pCO2 during reproductive conditioning would further increase the resilience of their larvae to elevated pCO2 or have negative consequences.
While early development of O. angasi larvae on the gills of adults (O’Sullivan 1978; Hickman and O’Meley 1988) in elevated pCO2 conditions relative to surrounding water may have provided resilience to detrimental effects of acidification, the presence of additional stressors (temperature, hyposalinity, and reduced food availability, which will occur in the real world, will likely minimise any advantage of O. angasi larvae to elevated pCO2. When fed the half food diet, development was slower and the percentage of abnormally shaped larvae was greater under elevated pCO2. The presence of other stressors exacerbated the effects of elevated pCO2 on decreased larval size. Moreover, elevated pCO2 as a single stressor negatively impacted clearance rates of larvae. Ocean acidification is believed to cause a higher energetic cost of living (Pörtner and Farrell 2008; Melzner et al. 2009). Many mollusc species have higher metabolic needs under elevated pCO2, with more energy being diverted to sustain processes such as acid–base balance and calcification (Melzner et al. 2009). It is hypothesised that at higher food concentrations, these higher metabolic costs can be met, thereby minimising the effects of elevated pCO2 (Pörtner and Farrell 2008; Melzner et al. 2011; Thomsen et al. 2013). At lower food concentrations, the higher costs cannot be met, exacerbating the impacts of elevated pCO2 (Pörtner and Farrell 2008). In the present study, we found that larvae of O. angasi decreased their feeding rate under elevated pCO2. Under high food concentrations, larvae reared under elevated pCO2 did not consume the food that they encountered as successfully as larvae reared in ambient conditions. Similarly, Vargas et al. (2013) found a decrease in feeding by larvae of the gastropod, Concholepas concholepas, with elevated pCO2 and highlighted the susceptibility of larval feeding ability to ocean acidification. Whether the larvae of O. angasi were unable to feed or had slowed metabolism as a defence mechanism is unknown. It is expected that the observed clearance rates by O. angasi larvae would be lower under low food concentrations because larvae encounter food less frequently (e.g. Tenore and Dunstan 1973; Widdows et al. 1979; Bayne 1993). Despite reduced feeding coupled with higher energetic requirements to deal with stress, over the longer term, larvae may ultimately survive if there is enough food available. These reduced clearance rates seen here as a sublethal effect are likely to ultimately compromise development and survival. Moreover, metabolic costs, not met by food intake, can lead to elevated stress which will ultimately lead to mortality (Pörtner 2008; Pörtner and Farrell 2008). It should also be noted that the positive impacts of food in oysters are limited with respect to minimising the negative impacts of ocean acidification (Hettinger et al. 2013).
Elevated pCO2 in combination with elevated temperature (30 °C) or decreased salinity (20) resulted in was a greater negative effect of acidification on larval development, abnormalities, and clearance rates. Bivalve development is well known to be sensitive to warming and hyposalinity (Przeslawski et al. 2015). As found here, juvenile clams (M. mercenaria) showed the greatest negative effects on biomineralisation and energy metabolism in conditions of low salinity and high pCO2 (700–800 μatm), while these stressors in isolation had no effects (Dickinson et al. 2013). Similarly, Ko et al. (2014) found that elevated pCO2, elevated temperature, and reduced salinity reduced pre-settlement growth of C. gigas, while these stressors used in isolation had no significant effects. Negative interactive effects of multiple climate stressors have implications for the larvae of O. angasi and other marine species as climate change is likely to result in exposure to many stressors with synergistic impacts leading to widespread changes to marine systems (Fabry et al. 2008; Przeslawski et al. 2015).
The interaction between temperature and salinity had a negative effect on the size and mortality of larvae and, independently, these stressors affected clearance rates. In ambient salinity, growth was faster at 30 °C than at 26 °C. In reduced salinity, the pattern was reversed. On average, over 50 % of larvae died under elevated temperature and reduced salinity, regardless of the level of pCO2 or food availability. The ability of larvae to clear food from the water column was limited when reared under elevated temperature or reduced salinity. Salinity is an important determinant of the distribution of oysters and their larvae (Dekshenieks et al. 2000). As a coastal species, O. angasi may be capable of withstanding some variation in salinity but are considered to inhabit a stenohaline environment (Thomson 1954; Nell and Gibbs 1986). The salinity of tidal estuaries where the larvae occur can drop to 20 for a number of days (Roy et al. 2001), which resulted only small effects on growth of O. angasi larvae level that reduces (O’Connor et al. 2014). Another estuarine oyster, S. glomerata, also shows low rates of growth and development at salinities of 20 or less (Dove and O’Connor 2007). Although the larvae were able to survive with reduced salinity at the optimal temperature of 26 °C, when temperature increased to 30 °C, fewer survived.
This study reared the larvae to the eyed stage. Taking the larvae to metamorphosis might have revealed differential mortality because stress in early life stages can influence the extent and magnitude of latent effects at later stages of development (Pechenik 2006. Stresses experienced by oysters in an early life stage can have negative “carry-over” to subsequent stages Hettinger et al. (2012). Larvae of O. lurida reared under ocean acidification conditions displayed decreased growth as juveniles regardless of their present environment (Hettinger et al. 2012). Naturally elevated levels of pCO2 had delayed effects on larval development of C. gigas, such that conditions affected mid-stage growth but not the early D-stage (Barton et al. 2012). In addition to carry-over effects within the life of individuals, oysters such as S. glomerata may carry responses to elevated pCO2 to their offspring and future generations (Parker et al. 2012, 2015).
In summary, O. angasi was affected by multiple climate change-related stressors, indicating that the impacts of climate change on O. angasi will be complex. Based on the findings of the present study, we predict that survival of O. angasi will continue in a changing climate, facilitated by its brooding life history. Further research is required to investigate climate change impacts on larval ontogeny and the effects on future generations in the long term. The results from this study suggest a complex interaction of pCO2, temperature, salinity, and availability of food on the growth, shape, development, and mortality of larvae. This study fills a vital gap in our understanding of the response of marine invertebrates to a changing climate, particularly when organisms are faced with multiple stressors (Harley et al. 2006). Due to preconditioning of larvae while brooded, the larvae of O. angasi may potentially have an improved capacity to compensate for changes to their acid–base status (Pörtner 2008). Without investigating the physiological mechanisms, it is not known whether larval preconditioning within the maternal shells, and ability to withstand experimentally created ocean acidification, will enable O. angasi to adapt to a high CO2 world in the future. It is essential for future studies to address multiple stressors associated with climate change (Byrne 2011; Przeslawski et al. 2015).
References
Barros P, Sobral P, Range P, Chicharo L, Matias D (2013) Effects of sea-water acidification on fertilization and larval development of the oyster Crassostrea gigas. J Exp Mar Biol Ecol 440:200–206
Barton A, Hales B, Waldbusser GG, Langdon C, Feely RA (2012) The Pacific oyster, Crassostrea gigas, shows negative correlation to naturally elevated carbon dioxide levels: implications for near-term ocean acidification effects. Limnol Oceangr 57:698–710
Bayne BL (1993) Feeding physiology of bivalves: time-dependence and compensation for changes in food availability Bivalve filter feeders. Springer, Berlin, Heidelberg, pp 1–24
Butler J (1982) Carbon dioxide equilibria and their applications. Addison-Wesley Publishing Company, New York
Butt D, O’Connor SJ, Kuchel R, O’Connor WA, Raftos DA (2008) Effects of the muscle relaxant, magnesium chloride, on the Sydney rock oyster (Saccostrea glomerata). Aquaculture 275:342–346
Byrne M (2011) Impact of ocean warming and ocean acidification on marine invertebrate life history stafes: vulnerabilities and potential for persistence in a changing ocean. In: Gibson RN, Atkinson RJA, Gordon JDM (eds) Oceanogr Mar Biol: Ann Rev, Vol 49, pp 1–42
Byrne M, Przeslawski R (2013) Multistressor impacts of warming and acidification of the ocean on marine invertebrates’ life histories. Integr Comp Biol 53:582–596
Byrne M, Ho M, Selvakumaraswamy P, Nguyen HD, Dworjanyn SA, Davis AR (2009) Temperature, but not pH, compromises sea urchin fertilization and early development under near-future climate change scenarios. Proc R Soc B Biol Sci 276:1883–1888
Byrne M, Gonzalez-Bernat M, Doo S, Foo S, Soars N, Lamare M (2013) Effects of ocean warming and acidification on embryos and non-calcifying larvae of the invasive sea star Patiriella regularis. Mar Ecol Prog Ser 473:235–246
Chaparro O, Cubillos V, Montiel Y, Paschke K, Pechenik J (2008) Embryonic encapsulation and maternal incubation: requirements for survival of the early stages of the estuarine gastropod Crepipatella dilatata. J Exp Mar Biol Ecol 365:38–45
Chaparro O, Segura C, Montory J, Navarro J, Pechenik J (2009) Brood chamber isolation during salinity stress in two estuarine mollusk species: from a protective nursery to a dangerous prison. Mar Ecol Prog Ser 374:145–155
Coughlan J (1969) The estimation of filtering rate from the clearance of suspensions. Mar Biol 2:356–358
Crenshaw MA (1972) The inorganic composition of molluscan extrapallial fluid. Biol Bull 143:506–512
Dekshenieks MM, Hofmann EE, Klinck JM, Powell EN (2000) Quantifying the effects of environmental change on an oyster population: a modeling study. Estuaries 23:593–610
Dickinson GH, Ivanina AV, Matoo OB, Pörtner HO, Lannig G, Bock C, Beniash E, Sokolova IM (2012) Interactive effects of salinity and elevated CO2 levels on juvenile eastern oysters, Crassostrea virginica. J Exp Biol 215:29–43
Dickinson GH, Matoo OB, Tourek RT, Sokolova IM, Beniash E (2013) Environmental salinity modulates the effects of elevated CO2 levels on juvenile hard-shell clams, Mercenaria mercenaria. J Exp Biol 216:2607–2618
Dix T (1976) Laboratory rearing of larval Ostrea angasi in Tasmania, Australia. J Malacol Soc Aust 3:209–214
Doney SC, Fabry VJ, Feely RA, Kleypas JA (2009) Ocean acidification: the other CO2 problem. Annu Rev Mar Sci 1:169–192
Dove MC, O’Connor WA (2007) Salinity and temperature tolerance of Sydney rock oysters Saccostrea glomerata during early ontogeny. J Shellfish Res 26:939–947
Dupont S, Dorey N, Stumpp M, Melzner F, Thorndyke M (2013) Long-term and trans-life-cycle effects of exposure to ocean acidification in the green sea urchin Strongylocentrotus droebachiensis. Mar Biol 160:1835–1843
Fabry VJ, Seibel BA, Feely RA, Orr JC (2008) Impacts of ocean acidification on marine fauna and ecosystem processes. ICES J Mar Sci 65:414–432
Feely RA, Sabine CL, Lee K, Berelson W, Kleypas J, Fabry VJ, Millero FJ (2004) Impact of anthropogenic CO2 on the CaCO3 system in the oceans. Science 305:362–366
Gazeau F, Quiblier C, Jansen JM, Gattuso JP, Middelburg JJ, Heip CHR (2007) Impact of elevated CO2 on shellfish calcification. Geophys Res Lett. doi:10.1029/2006gl028554
Gazeau F, Parker LM, Comeau S, Gattuso J-P, O’Connor WA, Martin S, Pörtner H-O, Ross PM (2013) Impacts of ocean acidification on marine shelled molluscs. Mar Biol 160:2207–2245
Gibson R, Atkinson R, Gordon J, Smith I, Hughes D (2011) Impact of ocean warming and ocean acidification on marine invertebrate life history stages: vulnerabilities and potential for persistence in a changing ocean. Oceanogr Mar Biol Annu Rev 49:1–42
Gobler CJ, Talmage SC (2013) Short- and long-term consequences of larval stage exposure to constantly and ephemerally elevated carbon dioxide for marine bivalve populations. Biogeosciences 10:2241–2253
Gobler CJ, DePasquale EL, Griffith AW, Baumann H (2014) Hypoxia and acidification have additive and synergistic negative effects on the growth, survival, and metamorphosis of early life stage bivalves. PLoS ONE. doi:10.1371/journal.pone.0083648
Gran G (1952) Determination of the equivalence point in potentiometric titrations—Part II. The Analyst 77:661–671
Guillard R (1975) Culture of phytoplankton for feeding marine invertebrates, culture of marine invertebrate animals. Springer, New York, pp 29–60
Harley CD, Randall Hughes A, Hultgren KM, Miner BG, Sorte CJ, Thornber CS, Rodriguez LF, Tomanek L, Williams SL (2006) The impacts of climate change in coastal marine systems. Ecol Lett 9:228–241
Helmuth BAT, Hofmann GE (2001) Microhabitats, thermal heterogeneity, and patterns of physiological stress in the rocky intertidal zone. Biol Bull 201:374–384
Hettinger A, Sanford E, Hill TM, Russell AD, Sato KN, Hoey J, Forsch M, Page HN, Gaylord B (2012) Persistent carry-over effects of planktonic exposure to ocean acidification in the Olympia oyster. Ecology 93:2758–2768
Hettinger A, Sanford E, Hill TM, Hosfelt JD, Russell AD, Gaylord B (2013) The influence of food supply on the response of Olympia oyster larvae to ocean acidification. Biogeosciences 10:6629–6638
Hickman NJ, O’Meley CM (1988) Culture of Australian flat oysters Ostrea angasi in Victoria, Australia: hatchery and nursery production. Marine Science Laboratories, Victoria
Hiebenthal C, Philipp EER, Eisenhauer A, Wahl M (2013) Effects of seawater pCO2 and temperature on shell growth, shell stability, condition and cellular stress of Western Baltic Sea Mytilus edulis (L.) and Arctica islandica (L.). Mar Biol 160:2073–2087
His E, Robert R, Dinet A (1989) Combined effects of temperature and salinity on fed and starved larvae of the Mediterranean mussel Mytilus galloprovincialis and the Japanese oyster Crassostrea gigas. Mar Biol 100:455–463
IPCC (2013) Climate change 2013: the physical science basis. Contribution of working group I to the fifth assessment report of the intergovernmental panel on climate change, Cambridge, United Kingdom and New York, NY, USA
IPCC (2014) Summary for Policymakers. In: Field CB, Barros VR, Dokken DJ, Mach KJ, Mastrandrea MD, Bilir TE, Chatterjee M, Ebi KL, Estrada YO, Genova RC, Girma B, Kissel ES, Levy AN, MacCracken S, Mastrandrea PR, White LL (eds) Climate change 2014: Impacts, adaptation, and vulnerability. Part A: global and sectoral aspects. Contribution of working group II to the fifth assessment report of the intergovernmental panel on climate change. Cambridge University Press, Cambridge, pp 1–32
Jansson A, Norkko J, Norkko A (2013) Effects of reduced pH on Macoma balthica larvae from a system with naturally fluctuating pH-dynamics. PLoS ONE. doi:10.1371/journal.pone.0068198
Kinne O (1971) Salinity: animals-invertebrates. In: Kinne O (ed) Marine ecology I part 2. Wiley-Interscience, London, pp 821–874
Ko GW, Dineshram R, Campanati C, Chan VB, Havenhand J, Thiyagarajan V (2014) Interactive effects of ocean acidification, elevated temperature, and reduced salinity on early-life stages of the Pacific Oyster. Environ Sci Technol 48:10079–10088
Kroeker KJ, Kordas RL, Crim RN, Singh GG (2010) Meta-analysis reveals negative yet variable effects of ocean acidification on marine organisms. Ecol Lett 13:1419–1434
Kroeker KJ, Kordas RL, Crim R, Hendriks IE, Ramajo L, Singh GS, Duarte CM, Gattuso JP (2013) Impacts of ocean acidification on marine organisms: quantifying sensitivities and interaction with warming. Glob Change Biol 19:1884–1896
Kroeker KJ, Gaylord B, Hill TM, Hosfelt JD, Miller SH, Sanford E (2014) The role of temperature in determining species’ vulnerability to ocean acidification: a case study using Mytilus galloprovincialis. PLoS ONE 9:e100353
Kurihara H (2008) Effects of CO2-driven ocean acidification on the early developmental stages of invertebrates. Mar Ecol Prog Ser 373:275–284
Kurihara H, Kato S, Ishimatsu A (2007) Effects of increased seawater pCO(2) on early development of the oyster Crassostrea gigas. Aquat Biol 1:91–98
Kurihara H, Asai T, Kato S, Ishimatsu A (2009) Effects of elevated pCO2 on early development in the mussel Mytilus galloprovincialis. Aquat Biol 4:225–233
Lewis E, Wallace D (1998) Program developed for CO2 system calculations. ORNL/CDIAC-105. Carbon dioxide Information Analysis Center, Oak Ridge National, Laboratory, US Department of Energy, Oak Ridge, TN
Mackenzie CL, Ormondroyd GA, Curling SF, Ball RJ, Whiteley NM, Malham SK (2014) Ocean warming, more than acidification, reduces shell strength in a commercial shellfish species during food limitation. PLoS ONE 9:e86764
Mardones-Toledo DA, Montory JA, Joyce A, Thompson RJ, Diederich CM, Pechenik JA, Mardones ML, Chaparro OR (2015) Brooding in the Chilean oyster Ostrea chilensis: unexpected complexity in the movements of brooded offspring within the mantle cavity. PLoS ONE 10:e0122859
Matoo OB, Ivanina AV, Ullstad C, Beniash E, Sokolova IM (2013) Interactive effects of elevated temperature and CO2 levels on metabolism and oxidative stress in two common marine bivalves (Crassostrea virginica and Mercenaria mercenaria). Comp Biochem Physiol A Mol Integr Physiol 164:545–553
Mehrbach C, Culberson C, Hawley J, Pytkowicz R (1973) Measurement of apparent dissociation constants of carbonic acid in seawater at atmospheric pressure. Limnol Oceanogr 18:897–907
Melzner F, Gutowska M, Langenbuch M, Dupont S, Lucassen M, Thorndyke M, Bleich M, Pörtner H-O (2009) Physiological basis for high CO2 tolerance in marine ectothermic animals: pre-adaptation through lifestyle and ontogeny? Biogeosciences 6:2313–2331
Melzner F, Stange P, Truebenbach K, Thomsen J, Casties I, Panknin U, Gorb SN, Gutowska MA (2011) Food supply and seawater pCO2 impact calcification and internal shell dissolution in the blue mussel Mytilus edulis. PLoS ONE. doi:10.1371/journal.pone.0024223
Munguia P, Alenius B (2013) The role of preconditioning in ocean acidification experiments: a test with the intertidal isopod Paradella dianae. Mar Freshw Behav Physiol 46:33–44
Nell J, Gibbs P (1986) Salinity tolerance and absorption of l-methionine by some Australian bivalves. Aust J Mar Freshw Res 37:721–727
Nguyen HD, Byrne M (2014) Early benthic juvenile Parvulastra exigua (Asteroidea) are tolerant to extreme acidification and warming in its intertidal habitat. Mar Ecol Prog Ser 453:36–42
Noisette F, Comtet T, Legrand E, Bordeyne F, Davoult D, Martin S (2014) Does encapsulation protect embryos from the effects of ocean acidification? the example of Crepidula fornicata. PLoS ONE 9:e93021
O’Sullivan B (1978) The fertility of the Port Lincoln oyster (Ostrea angasi, Sowerby) from the West Lakes, South Australia. Aquac Asia 19:11
O’Connor WA, Dove MC (2009) The changing face of oyster culture in New South Wales, Australia. J Shellfish Res 28:803–811
O’Connor S, Moltschaniwskyj NA, Bolch C, O’Connor WA (2012) Dietary influence on growth and development of flat oyster, Ostrea angasi (Sowerby, 1871) larvae. Aquac Res 43:1317–1327
O’Connor S, Moltschaniwskyj NA, O’Connor WA (2014) Assessment of temperature or salinity effects on larval development by catecholamine induced metamorphosis of hatchery reared flat oyster, Ostrea angasi (Sowerby 1871) larvae. Aquac Res. doi:10.1111/are.12408
Orr JC, Fabry VJ, Aumont O, Bopp L, Doney SC, Feely RA, Gnanadesikan A, Gruber N, Ishida A, Joos F, Key RM, Lindsay K, Maier-Reimer E, Matear R, Monfray P, Mouchet A, Najjar RG, Plattner GK, Rodgers KB, Sabine CL, Sarmiento JL, Schlitzer R, Slater RD, Totterdell IJ, Weirig MF, Yamanaka Y, Yool A (2005) Anthropogenic ocean acidification over the twenty-first century and its impact on calcifying organisms. Nature 437:681–686. doi:10.1038/nature04095
Parker L, Ross P, O’Connor W (2009) The effect of ocean acidification and temperature on the fertilisation and embryonic development of the Sydney rock oyster Saccostrea glomerata (Gould 1850). Glob Change Biol 15:2123–2136
Parker L, Ross P, O’Connor W (2010) Comparing the effect of elevated pCO2 and temperature on the fertilization and early development of two species of oysters. Mar Biol 157:2435–2452
Parker LM, Ross PM, O’Connor WA (2011) Populations of the Sydney rock oyster, Saccostrea glomerata, vary in response to ocean acidification. Mar Biol 158:689–697
Parker LM, Ross PM, O’Connor WA, Borysko L, Raftos DA, Pörtner HO (2012) Adult exposure influences offspring response to ocean acidification in oysters. Glob Change Biol 18:82–92
Parker LM, Ross PM, O'Connor WA, Pörtner HO, Scanes E, Wright JM (2013) Predicting the response of molluscs to the impact of ocean acidification. Biol 2:651–692
Parker LM, O’Connor WA, Raftos DA, Pörtner H-O, Ross PM (2015) Persistence of positive carryover effects in the Oyster, Saccostrea glomerata, following transgenerational exposure to ocean acidification. PLoS ONE 10:e0132276
Pechenik JA (2006) Larval experience and latent effects—metamorphosis is not a new beginning. Integr Comp Biol 46:323–333
Pedersen E, Perkins M (1986) The use of benthic invertebrate data for evaluating impacts of urban runoff. Hydrobiologia 139:13–22
Pörtner H-O (2008) Ecosystem effects of ocean acidification in times of ocean warming: a physiologist’s view. Mar Ecol Prog Ser 373:203–217
Pörtner H-O, Farrell AP (2008) Ecology physiology and climate change. Science 322:690–692
Przeslawski R, Byrne M, Mellin C (2015) A review and meta-analysis of the effects of multiple abiotic stressors on marine embryos and larvae. Glob Change Biol 21:2122–2140
Ries JB, Cohen AL, McCorkle DC (2009) Marine calcifiers exhibit mixed responses to CO2-induced ocean acidification. Geology 37:1131–1134
Robert R, His E, Dinet A (1988) Combined effects of temperature and salinity on fed and starved larvae of the European flat oyster Ostrea edulis. Mar Biol 97:95–100
Ross PM, Parker L, O’Connor WA, Bailey EA (2011) The impact of ocean acidification on reproduction, early development and settlement of marine organisms. Water 3:1005–1030
Roy PS, Williams RJ, Jones AR, Yassini I, Gibbs PJ, Coates B, West RJ, Scanes PR, Hudson JP, Nicholi S (2001) Structure and function of south-east Australian estuaries. Estuar Coast Shelf Sci 53:351–384
Sanders MB, Bean TP, Hutchinson TH, Le Quesne WJF (2013) Juvenile king scallop, Pecten maximus, is potentially tolerant to low levels of ocean acidification when food is unrestricted. PLoS ONE. doi:10.1371/journal.pone.0074118
Scanes E, Parker LM, O’Connor WA, Ross PM (2014) Mixed effects of elevated pCO2 on fertilisation, larval and juvenile development and adult responses in the mobile subtidal scallop Mimachlamys asperrima (Lamarck, 1819). PLoS ONE 9:e93649
Schone BR, Tanabe K, Dettman DL, Sato S (2003) Environmental controls on shell growth rates and delta O-18 of the shallow-marine bivalve mollusk Phacosoma japonicum in Japan. Mar Biol 142:473–485
Sheppard-Brennard H, Dworjanyn SN, Davis AR, Byrne M (2010) Impact of ocean warming and ocean acidification on larval development and calcification in the sea urchin Tripneustes gratilla. PLoS ONE 5(6):e11372. doi:10.1371/journal.pone.0011372
Talmage SC, Gobler CJ (2011) Effects of elevated temperature and carbon dioxide on the growth and survival of larvae and juveniles of three species of northwest atlantic bivalves. PLoS ONE. doi:10.1371/journal.pone.0026941
Tenore K, Dunstan W (1973) Comparison of feeding and biodeposition of three bivalves at different food levels. Mar Biol 21:190–195
Thomsen J, Gutowska M, Saphörster J, Heinemann A, Trübenbach K, Fietzke J, Hiebenthal C, Eisenhauer A, Körtzinger A, Wahl M (2010) Calcifying invertebrates succeed in a naturally CO2-rich coastal habitat but are threatened by high levels of future acidification. Biogeosciences 7:3879–3891
Thomsen J, Casties I, Pansch C, Koertzinger A, Melzner F (2013) Food availability outweighs ocean acidification effects in juvenile Mytilus edulis: laboratory and field experiments. Glob Change Biol 19:1017–1027
Thomson J (1954) The genera of oysters and the Australian species. Mar Freshw Res 5:132–179
Underwood AJ (1997) Ecological experiments: their logical design and interpretation using analysis of variance. Cambridge University Press, Melbourne
Underwood AJ, Chapman MG (2002) GMAV5 for windows. Institute of Marine Ecology, The University of Sydney, Sydney
Van Colen C, Debusschere E, Braeckman U, Van Gansbeke D, Vincx M (2012) The early life history of the clam Macoma balthica in a high CO2 world. PLoS ONE. doi:10.1371/journal.pone.0044655
Vargas CA, de la Hoz M, Aguilera V, San Martín V, Manríquez PH, Navarro JM, Torres R, Lardies MA, Lagos NA (2013) CO2-driven ocean acidification reduces larval feeding efficiency and changes food selectivity in the mollusk Concholepas concholepas. J Plank Res 35:1059–1068
Waldbusser GG, Voigt EP, Bergschneider H, Green MA, Newell RI (2011) Biocalcification in the eastern oyster (Crassostrea virginica) in relation to long-term trends in Chesapeake Bay pH. Estuaries Coasts 34:221–231
Waldbusser G, Hales B, Langdon C, Haley B, Schrader P, Brunner E, Gray M, Miller C, Gimenez I (2015a) Saturation-state sensitivity of marine bivalve larvae to ocean acidification. Nat Clim Change 5:273–280
Waldbusser GG, Hales B, Langdon CJ, Haley BA, Schrader P, Brunner EL, Gray MW, Miller CA, Gimenez I, Hutchinson G (2015b) Ocean acidification has multiple modes of action on bivalve larvae. PLoS ONE 10:e0128376
White MM, McCorkle DC, Mullineaux LS, Cohen AL (2013) Early exposure of bay scallops (Argopecten irradians) to high CO2 causes a decrease in larval shell growth. PLoS ONE. doi:10.1371/journal.pone.0061065
Widdows J, Fieth P, Worrall C (1979) Relationships between seston, available food and feeding activity in the common mussel Mytilus edulis. Mar Biol 50:195–207
Wood HL, Spicer JI, Widdicombe S (2008) Ocean acidification may increase calcification rates, but at a cost. Proc R Soc B Biol Sci 275:1767–1773
Zimmerman KM, Pechenik JA (1991) How do temperature and salinity affect relative rates of growth, morphological-differentiation, and time to metamorphic competence in larvae of the marine gastropod Crepidula plana. Biol Bull 180:372–386
Acknowledgments
The authors acknowledge assistance from J. Wright (University of Western Sydney) and the staff at Port Stephens Fisheries Institute, B. Archer, K. Johnston, and A. Parnell. Funding was provided by an internal grant from the University of Western Sydney Research Grant Scheme (Science, Technology, Engineering and Medicine) Program (LMP and PMR).
Author information
Authors and Affiliations
Corresponding author
Additional information
Responsible Editor: H. Pörtner.
Reviewed by F. Gazeau and an undisclosed expert.
Rights and permissions
About this article
Cite this article
Cole, V.J., Parker, L.M., O’Connor, S.J. et al. Effects of multiple climate change stressors: ocean acidification interacts with warming, hyposalinity, and low food supply on the larvae of the brooding flat oyster Ostrea angasi . Mar Biol 163, 125 (2016). https://doi.org/10.1007/s00227-016-2880-4
Received:
Accepted:
Published:
DOI: https://doi.org/10.1007/s00227-016-2880-4