Abstract
BLB1 is a new Bacillus thuringiensis kurstaki strain, isolated from a Tunisian soil sample. Assay of toxicity of BLB1 crystal proteins resulted in an LC50 of 70.32 ng of toxin per mg of flour against third instar Ephestia kuehniella with confidence limits of (31.6–109.04 ng). This LC50 is less than that of the commercial strains HD1 used as a reference. The characterization of this strain by scanning transmission electron microscopy, analysis of its cry genes content by PCR-sequencing, and analysis of its δ-endotoxin patterns demonstrate that it belongs to the same subgroup than HD1, but ruled out the involvement of cry gene content or protoxin activation in the hypertoxicity of this strain. Taking into account the δ-endotoxin/spore ratio for each strain, and by allowing the estimation of the production level per spore, it might be concluded that BLB1 production is the highest, when compared with that of HD1. On the basis of its toxicity, BLB1 could be considered as a strain of great interest and would allow the production of quantities of bioinsecticides at low cost.
Similar content being viewed by others
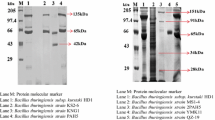
Avoid common mistakes on your manuscript.
Introduction
Bacillus thuringiensis is an aerobic, spore-forming, gram-positive bacterium that synthesizes crystalline proteins during sporulation (Bechtel and Bulla 1976). These proteins are specifically toxic to insect larvae and are widely used as bioinsecticides against lepidopteran, dipteran, and coleopteran pests. Crystal proteins from numerous strains have been classified according to the similarity of their amino acid sequences and their insecticidal specificity (Höfte and Whiteley 1989).
In general, most Lepidopteron-specific B. thuringiensis toxins are known to be synthesized as a protein crystals composed of protoxin molecules of 130–140 kDa which, upon ingestion by larvae of a susceptible species, are dissolved by alkaline midgut fluid and proteolytically processed to an active toxin of 60 kDa. Subsequently, the active toxin binds to specific receptors on the surface of midgut epithelial cells, followed by the insertion of the hydrophobic region of the toxin molecule into the cell membrane and formation of a transmembrane pore, which eventually results in cell lysis. Disruption of the gut epithelium leads to starvation, septicaemia, and ultimately to death of the intoxicated larvae. Gut protease–toxin interactions are complex and poorly understood (Rouis et al. 2007; 2008). The toxin’s secondary structure and the toxin receptors on insect midgut epithelial cells are important determinants of toxin specificity (Knowles 1994; Masson et al. 1994; Schwartz et al. 1997) and it is likely that even a modest amount of overdigestion may alter toxin specificity or alternatively, impair binding of the toxin to its receptor resulting in loss of activity (Pang and Gringorten 1998). Indeed, it has been shown that differential protoxin proteolysis by the gut juice of different larvae can effect toxicity.
For decades, worldwide screening followed by isolation and characterization of new B. thuringiensis strains has been carried out to discover strains with novel or particularly high insecticidal activities (Bravo et al. 1998; Ibarra et al. 2003; Martínez et al. 2005; Monnerat et al. 2007).
In the present work, we describe the novel interesting B. thuringiensis kurstaki strain named BLB1. The latter was isolated from Tunisian soil sample and identified by ARDRA and by genomic DNA RFLP pulse field gel electrophoresis (PFGE) as B. thuringiensis kurstaki showing a very high insecticidal activity against Lepidoptera, compared to hundreds of B. thuringiensis strains isolated in Tunisia. The purified BLB1 crystals were subjected to electron microscopy observation, and their δ-endotoxin content and activation were studied. On the other hand, BLB1 cry genes type and copy numbers were determined by PCR-sequencing and real time PCR.
Materials and methods
Bacterial strains and growth conditions
BLB1 was a B. thuringiensis strain newly isolated in our laboratory. B. thuringiensis subsp. kurstaki HD1 (Carozzi et al. 1991) was used as reference strain. Luria-Bertani (LB) (Sambrook et al. 1989) and T3 (Travers et al. 1987) culture media were used for the growth of B. thuringiensis strains in order to, respectively, extract DNA and follow the parasporal crystal formation during growth.
Chromosomal DNA extraction, restriction endonuclease cleavage, and PFGE
Chromosomal DNA was extracted by the technique of Herrel et al. (1995); for restriction endonuclease cleavage, 3 mm slices of agarose embedded samples plugs were cleaved with NotI and SmaI enzymes according to the manufacturer’s recommendations (biotools, Spain). Samples were first equilibrated in the recommended restriction buffer for several hours at the optimal temperature of each enzyme (NotI, 37°C and SmaI, 25°C). Then fresh restriction buffer and 20 U of enzyme were added to plugs and incubated overnight.
Cleaved plugs were loaded onto a 1% agarose gel [pulsed field certified agarose (Bio-Rad) prepared in 0.5× Tris borate–EDTA running buffer) and electrophoresis was performed with the Bio-Rad CHEF-DRII system. Electrophoresis was run at 6 V/cm and 14°C with ramping from 5.3 to 66 s over 19.5 h for SmaI, but for NotI the running condition consists on two blocks: 4–8 s for 10 h and 8–40 s for 11 s at 6 V/cm and 14°C.
ARDRA analysis of PCR amplicons
16S rDNA fragments were amplified using a 19-mer forward primer (B-K1/F, 5′-TCACCAAGGCRACGATGCG-3′) and a 18-mer reverse primer (B-K1/R1, 5′CGTATTCACCGCGGCATG-3′) designed by Yang et al. (2006). The ARDRA technique is performed using five restriction enzymes: TaqI, MspI; MboII, and AluI, having 1, 2, 3, 4, and 5 recognition sites, respectively, based on the sequence of the B. thuringiensis 16s rDNA retrieved from Gen-Bank. PCR products were purified using WIZARD PCR purification columns (Promega, Australia) and were eluted in a final volume of 40 μl. A 5-μl sample of purified PCR amplicon was digested with 5 U of individual restriction enzyme in a 20-μl reaction volume for 4 h using the conditions recommended by the manufacturer. The restriction fragments were electrophoresed through a 2% agarose gel followed by ethidium bromide staining.
Bioassays
Bioassays were carried out using third instar Ephestia kuehniella, kindly provided by the Institute of Olive Tree, Sfax, Tunisia, as described by Tounsi et al. (2005). Fifty percent lethal concentration (LC50) for each strain was calculated after 48 h, from pooled raw data by probit analysis using programs written in the R. language (Venables and Smith 2004). The control for bioassays was performed using the B. thuringiensis strain HD1.
Electron microscopy
The spore-crystal mixture was deposited on a carbon-coated electro microscopy grid and stained with uranyl acetate. Images were recorded using a Transmission Electron microscope (CM120- Philips), operating at 100 kv, on a CCD camera.
Bioinsecticide production
Bacillus thuringiensis strains were grown in the liquid medium described by Zouari et al. (1998). This medium contains 15 g/l glucose, 5.4 g/l ammonium sulphate, 5 g/l yeast extract, and 5 g/l glycerol. The following minerals were used (g/l): KH2PO4, 1; K2HPO4, 1; MgSO4, 0.3; MnSO4, 0.01; and FeSO4, 0.01. The pH was adjusted to 7 before sterilization. In each shaker flask, 1 g of CaCO3 was added for pH stabilization. Flasks, containing 50 ml of culture medium, were incubated 72 h at 30°C and 200 rpm in a rotary shaker.
PCR amplification and DNA sequencing
A number of previously described primers, listed in Table 1, were used in the PCR analysis (Jaoua et al. 1996) to identify known cry-type genes present in the strain BLB1. PCR assays were performed in a reaction mixture containing 20 ng of B. thuringiensis chromosomal DNA, 10 mM Tris–HCl, 50 mM KCl, 400 μM of each dNTP, 0.2 μM each primer, 2.5 mM MgCl2, 1.25 UI Taq polymerase (Pharmacia, Vienna, Austria). Thermal cycler conditions consisted of a single denaturation step (3 min at 94°C) followed by the 35-cycle program with an initial denaturation at 94°C for 45 s. For all the primers used, the PCR annealing temperature was 50°C and extension was performed at 72°C for 1 min and 30 s. PCR fragments were analyzed by submarine gel electrophoresis (Sambrook et al. 1989). Expected-size fragments were excised and purified by the Wizard PCR Clean up system (Promega Inc., Madison, WI, USA), and their respective identity confirmed by direct sequencing using an ABI model 3100 automated sequencer (Applied Biosystems, USA). The procedures for fragments’ purification from gels and dye-terminator cycle-sequencing followed the manufacturers’ recommendations.
Qualitative PCR conditions
The qualitative PCR assay was performed using 100 ng of genomic DNA as template in a volume of 25 μl. The reaction mixture contained 1× PCR buffer (10 mM Tris–HCl, pH 8.3, 50 mM KCl), 200 μM of each dNTPs, 2.5 mM MgCl2, and 1 unit Hot Start Taq (Biotools, Spain). The PCR amplifications were carried out on a GeneAmp_9700 thermal cycler using the following program: a 94°C initial denaturation step for 5 min; followed by 40 cycles of 94°C denaturation for 30 s, 55.9°C annealing for 30 s, and 72°C extension for 30 s; and a 72°C final extension for 10 min. For the cry1A genes, we used: CRY1AQL forward primer (5′ GCGACTATCAATAGTCGTTAT-3′) and CRY1AQR reverse primer (5′-CCAATCTCTAGAATCCGGTC-3′).
Solubilization, proteolysis assay and SDS-PAGE of δ-endotoxins
Total crystals (a mixture of bipyramidal and cuboidal crystals) from strains BLB1 and HD-1 were purified as reported by Lee et al. (2001) and treated with 0.05 M Na2CO3, pH 9.5, containing 0.01% β-mercaptoethanol for 30 min at 37°C. This procedure allows the solubilization of only the bipyramidal crystals. Solubilzed protoxins were incubated with soluble proteinase extract from E. kuenhniella third instar larvae at 1:50 (V/V) and commercial trypsin and chymotrypsin at different ratios in a final volume of 25 μl at room temperature with constant agitation for different incubation periods. Samples were separated by sodium dodecyl sulfate 10% polyacrylamide gel electrophoresis (SDS-PAGE). Proteins were visualized by coomassie blue staining.
Biomass and δ-endotoxin yield determination
Microscopically, we observed that after 72 h, the cultures were composed of a mixture of spores, crystals, and minor cell debris. The number of spores was estimated by the determination of colony-forming units (CFU), at the end of the experiments. Culture samples were heated at 80°C for 10 min and appropriate dilutions were plated on solid LB medium. δ-endotoxin concentration was determined as follows as described by Zouari et al. (1998), Zouari and Jaoua (1999) and Ghribi et al. (2007): 1 ml of culture medium was centrifuged for 10 min at 10,000g and the pellet was washed twice with 1 M NaCl and twice with distilled water. The pellet was then suspended in 1 ml of 50 mM NaOH (pH 12.5). After 3 h of incubation at 30°C, total protein in the supernatant was measured by using Bio-Rad reagent (Bio-Rad Protein assay, Cat. 500-0006). Because of the potential for contamination of spore–crystal mixtures by dissolved proteins, the strain HD1 was used as a reference. The yield of δ-endotoxin production was calculated as following: δ-endotoxin (mg/l) divided by CFU (spores/l). The data related to determination of δ-endotoxin production were the average of three replicates of two separate experiments for each cultural condition. They were statistically analyzed by using SPSS software (version 100; Duncan’s test performed after ANOVA).
Preparation of cry proteins for bioassays
Spore–crystal mixtures of each bacterial strain culture were centrifuged for 10 min at 10,000 rpm and the pellets were washed twice with 1 M NaCl and twice with distilled water and then lyophilized. δ-endotoxins in lyophilized spore–crystal mixtures were further solubilized in 50 mM NaOH and their concentrations were determined using Bio-Rad reagent (Bio-Rad Protein assay, Cat. 500-0006). The strain HD1 was used as a reference.
Results and discussion
Identification of B. thuringiensis BLB1 strain using ARDRA-ribotyping and pulse field gel electrophoresis of digested genome
In this study, we found that the PFGE of macrorestricted BLB1 and control kurstaki strain HD1 genomic DNA with SmaI and NotI (Fig. 1) showed clearly the same PFGE fingerprint, consisting a minimum of 22 bands ranging from 35 kb to 1.1 Mb (Fig. 1) suggesting that BLB1 is a B. thuringiensis kurstaki strain. The PFGE technique with SmaI for DNA macrorestriction is highly discriminatory and a reproducible method for bacteria typing (Liu and Wu 1997). This result was confirmed by the fact that BLB1 showed the same 16S rDNA ARDRA profile than HD1 using TaqI, AluI, MboII, and MspI restriction enzymes (data not shown).
BLB1 strain crystals ultrastructure analysis
The ultrastructure of the crystals from BLB1 was obtained by transmission electron microscopy. As shown in Fig. 2 two types of crystal morphology were observed: bipyramidal and cubic. The one with a bipyramidal shape is typically associated with Cry1 toxins whereas the cubic square crystal contains most probably Cry2 toxin (Höfte and Whiteley 1989). The enormous difference of shape between the two types is in agreement with the fact that this strain showed the highest lepidopteron activity. Indeed, as reported by Bobrowski et al. (2002), although the correlation between the type of crystal morphology and the level of insecticidal activity is not clear, several previous studies have confirmed that the more toxic strains to lepidopteran larvae form bipyramidal crystals.
BLB1 insecticidal activity investigation
In a previous study, bioassays were performed with third instar E. kuehniella larvae in order to identify the most potent strains and to allow their exploitation for the optimization of biological control programs. The B. thuringiensis strain BLB1, a novel B. thuringiensis isolate obtained from Tunisian soil sample, showed the highest activity against this larva in our laboratory collection.
When compared to the commercial strain HD1 (Table 1), assay of BLB1 crystal proteins resulted in an LC50 of 70 ng of toxin per mg of flour against E. kuehniella with confidence limits of (32–108 ng/mg). LC50 of HD1 was 137 ng of toxins per mg of flour with a confidence limit of (111–167 ng/mg). These results were obtained with a slope of 0.01. No mortality was observed in the negative control. These results show that LC50 of BLB1 is almost two times lower than that of HD1. The new strain exhibited a significantly greater potency than the standard strain HD1 against lepidopteron insect E. kuehniella. It was then applied for further analyses.
BLB1 cry gene content
One of the most important factors which could play a key role in this difference of toxicity is the cry genes content. In general, the type of cry genes present in a strain correlates in some extent with its insecticidal activity. Thus, the identification of the gene content in a strain can be used to predict or confirm its insecticidal potential. The presence of Cry1, reported to be active against Lepidopteron insects and Cry2 forming typical cuboidal crystals, was then checked in our new isolate. The kurstaki HD-1 strain was used as reference strain since harboring the cry1Aa, cry1Ac, cry1Ab, cry1Ia, and cry2 genes. The prediction of the presence of the genes cry1 and cry2 was done by PCR using primers designed by several authors (Table 2). PCR Fragments with expected sizes of 908, 986, 720, and 625 bp corresponding, respectively, to cry1Aa–cry1Ac, cry1Ab, cry1Ia, and cry2 were obtained (data not shown). All these fragments were sequenced in both directions and alignment with known cry genes (EMBL/Gen Bank) revealed identities of 100% with the last mentioned genes.
To estimate and compare the copy number of cry1A genes between BLB1 and HD1, real time quantitative PCR assays were performed using plasmid DNA as template and cry1A gene specific primers. HD1, HD1CryB, HD1CryBpHTblue-cry1Ac, and BNS3 strains were used as controls (Fig. 3). A clear difference in the threshold cycle (Ct) suggesting a quantitative variation of these genes. Indeed, Ct values are 22.7, 10.8, 10.06, 9.3, and 9.13 corresponding, respectively, to HD1 CryB, BNS3, HD1, HD1CryBpHTblue-cry1Ac, and BLB1 strains. The BLB1 cry1A copy number was significantly higher than HD1 strain one (P < 0.05) which could be one of the factors responsible of its hypertoxicity.
BLB1 protein profiles and δ-endotoxin proteolysis activation
As mentioned above, the difference of toxicity could be a result of the expression level or the difference in copy number of the cry1A genes contained in both strains. Protein profile of crystal protein extract of BLB1 strain was then analyzed and compared to that of HD1. Both profiles were similar and showed one band of around 130 kDa which supported that cry1 genes were expressed (Fig. 4). Another band corresponding to Cry2 protein (~70 kDa) was also observed.
The higher lepidopteron activity observed with the strain BLB1 could be associated with enhancement of one or more steps in the cascade of events involved in the mode of action of these δ-endotoxins (Ferré et al. 1995). The characterisation of this strain was then completed by the activation assays of the protoxin 130 kDa (containing the presumed products of cry1 genes) by midgut homogenate of E. kuehniella and commercial trypsin, and chymotrypsin at different times of incubation. HD1 was used as control. As shown in Fig. 5, there was no major difference in proteolytic patterns of BLB1 and HD1 in the three cases. However, it is interesting to notice that the observed profile in the case of chymotrypsin cleavage (Fig. 5c) was obtained by protoxine: protease ratio of 266:1. In the case of trypsin and using the same ratio, the stable toxin band was observed very early (5 min, data not shown) suggesting that the trypsin is more active on both toxin mixtures. Therefore, the assay was repeated with a lower concentration protease (Fig. 5b, ratio protoxine: protease ~2,000:1). On the other hand and when the three patterns were compared we observe that the one obtained by larvae homogenate was vary similar to that obtained by the trypsin, indicating the presence of high trypsin and low chymotrypsin levels in the midgut. The presence of predominant trypsin-like activity in the midgut homogenate of E. kuehniella has been also reported in many other insects (Christeller et al. 1992; Marchetti et al. 1998; Shao et al. 1998, Mohan and Gujar 2002). Therefore, our results ruled out the involvement of proteases activity in the enhancement of BLB1 toxicity compared to HD1. Extensive studies on the interaction of the BLB1 toxins with E. kuehniella midgut may reveal the relative importance of receptor binding or other factors in this toxicity.
SDS-PAGE analysis of the protoxin 130 kDa activation of BLB1 and HD1 with: a E. kuehniella midgut juice, b trypsin (ratio protoxine: protease ~2,000:1) and c chymotrypsin (ratio protoxine: protease 266:1). a, b lines 1–6 protoxin incubated with proteases for 15, 30, 60, 120 min, 6, and 13 h, respectively; for figure c lines 1–5 protoxin incubated with proteases for 15, 30, 120 min, 6, and 13 h, respectively. NC non cleaved, M protein molecular weight markers
Study of the BLB1 spore and δ-endotoxin production
On the basis of its toxicity, BLB1 is of a great importance from practical point of view since it could be possible to produce large quantities of bioinsecticides with less cost. That is why we look for the comparison of the δ-endotoxins production between this strain and the reference strain (HD1). Results are summarized in Table 3. A reduction of 40% of the final spore titres and an improvement of 178% in δ-endotoxin synthesis yield were obtained with BLB1 strain compared to HD1. Therefore, taking into account these results, it might be concluded that BLB1 production is the highest.
References
Bechtel DB, Bulla LA Jr (1976) Electron microscope study of sporulation and parasporal crystal formation in Bacillus thuringiensis. J Bacteriol 127:1472–1481
Bobrowski VL, Pscali G, Bodanese-zanetti MH, Fiuza LM (2002) Characterization of two Bacillus thuringiensis isolates from south Brasil and their toxicity against Anticarsia gemmatalis (Lepidoptera: Noctuidae). Biol Control 25:129–135
Bravo A, Sarabia S, Lopez L, Ontiveros H, Abarca C, Ortiz A, Ortiz M, Lina L, Villalobos FJ, Pena G, Nunez-Valdez ME, Soberon M, quintero R (1998) Characterization of cry genes in a Mexican Bacillus thuringiensis strain collection. Appl Environ Microbiol 64:4965–4972
Carozzi NB, Kramer VC, Warren GW, Evola S, Koziel M (1991) Prediction of insecticidal activity Bacillus thuringiensis strain by polymerase chain reaction product profiles. Appl Environ Microbiol 57:353–356
Christeller JT, Laing WA, Markwick NP, Burgess EPJ (1992) Midgut protease activities in 12 phytophagous lepidopteran larvae: dietary and protease inhibitor interactions. Insect Biochem Mol Biol 22:735–746
Ferré J, Escriche B, Bel Y, Van Rie J (1995) Biochemistry and genetics of insect resistance to Bacillus thuringiensis insecticidal crystal proteins. FEMS Microbiol Lett 132:1–7
Geiser M, Schweitzer S, Grimm C (1986) The hypervariable region in the genes coding for entomopathogenic crystal proteins of Bacillus thuringiensis: nucleotide sequence of the kur Hdl gene of subsp Kurstaki HD-1. Gene 48:109–118
Ghribi D, Zouari N, Trigui W, Jaoua S (2007) Use of sea water as salts source in starch- and soya bean-based media, for the production of Bacillus thuringiensis bioinsecticides. Process Biochem 42:374–378
Herrel LJ, Anderson GL, Wilson KH (1995) Genetic variability of Bacillus thuringiensis and related species. J Clin. Microbiol 33:1847–1850
Höfte H, Whiteley HR (1989) Insecticidal crystal proteins of Bacillus thuringiensis. Microbiol Rev 53:242–255
Ibarra JE, Del Rincón MC, Ordúz S, Noriega D, Benintende G, Monnerat R, Regis L, de Oliveira CMF, Lanz H, Rodriguez MH, Sánchez J, Peña G, Bravo A (2003) Diversity of Bacillus thuringiensis strains from Latin America with insecticidal activity. Appl Environ Microbiol 69:5269–5274
Jaoua S, Zouari N, Tounsi S, Ellouz R (1996) Study of the δ-endotoxins produced by three recently isolated strains of Bacillus thuringiensis. FEMS Microbiol Lett 145:349–354
Knowles BH (1994) Mechanism of action of Bacillus thuringiensis insecticidal delta-endotoxins. In: Evans PD (ed) Advances in insect physiology, vol 24. Academic Press, London, pp 275–308
Lee IH, Je YH, Chang JH (2001) Isolation and characterization of a Bacillus thuringiensis ssp. kurstaki strain toxic to Spodoptera exigua and Culex pipiens. Curr Microbiol 43:284–287
Liu PYF, Wu WL (1997) Use of different PCR-based DNA fingerprinting techniques and pulsed-field gel electrophoresis to investigate the epidemiology of Acinetobacter calcoaceticus–Acinetobacter baumannii complex. Diagn Microbiol Infect Dis 28:19–28
Marchetti S, Chiaba C, Chiesa F, Bandiera A, Pitotti A (1998) Isolation and partial characterization of two trypsins from the larval midgut of Spodoptera littoralis (Boisduval). Insect Biochem Mol Biol 28:449–458
Martínez C, Jorge E, Ibarra C, Primitivo C (2005) Association analysis between serotype, cry gene content, and toxicity to Helicoverpa armigera larvae among Bacillus thuringiensis isolates native to Spain. J Invertebr Pathol 90:91–97
Masson L, Mazza A, Gringorten L, Baines D, Aneliunas V, Brousseau R (1994) Specificity domain localization of Bacillus thuringiensis insecticidal toxins is highly dependent on the bioassay system. Mol Microbiol 14:851–860
Mohan M, Gujar GT (2002) Geographical variation in larval susceptibility of the diamondback moth, Plutella xylostella (Lepidoptera: Plutellidae) to Bacillus thuringiensis spore-crystal mixtures and purified crystal proteins, and associated resistance development in India. Bull Ent Res 92:489–498
Monnerat RG, Batista AC, Telles de Medeiros P, Martins ES, Melatti VM, Praça LB, Dumas VF, Morinaga C, Demo C, Gomes ACM, Falcão R, Siqueira CB, Silva-Werneck JO, Berry C (2007) Screening of Brazilian Bacillus thuringiensis isolates active against Spodoptera frugiperda, Plutella xylostella and Anticarsia gemmatalis. Biol Control 41:291–295
Pang ASD, Gringorten JL (1998) Degradation of Bacillus thuringiensis delta-endotoxin in host insect gut juice. FEMS Microbiol Lett 167:281–285
Rouis S, Chakroun M, Saadaoui I, Jaoua S (2007) Proteolysis, histopathological effects, and immunohistopathological localization of delta-endotoxins of Bacillus thuringiensis subsp. kurstaki in the midgut of lepidopteran olive tree pathogenic insect Prays oleae. Mol Biotechnol 35:141–148
Rouis S, Chakroun M, Jaou S (2008) Comparative study of Bacillus thuringiensis Cry1Aa and Cry1Ac δ-endotoxin activation, inactivation and in situ histopathological effect in Ephestia kuehniella (Lepidoptera: Pyralidae). Mol Biotechnol 38:233–239
Sambrook J, Frisch EF, Maniatis T (1989) Molecular Cloning: a laboratory manual, 2nd edn. Cold Spring Harbor Laboratory Press, Cold Spring Harbor
Schwartz JL, Juteau M, Grochulski P, Cygler M, Préfontaine G, Brousseau R, Masson L (1997) Restriction of intramolecular movements within the Cry1Aa toxin molecule of Bacillus thuringiensis through disulphide bond engineering. FEBS Lett 410:397–402
Shao Z, Cui Y, Liu X, Yi H, Ji J, Yu Z (1998) Processing of δ-endotoxin of Bacillus thuringiensis subsp. kurstaki HD-1 in Heliothis armigera midgut juice and the effects of protease inhibitors. J Invertebr Pathol 72:73–81
Shin BS, Park SH, Choi SK, Koo BT, Lee ST, Kim JI (1995) Distribution of cryV-type insecticidal protein genes in Bacillus thuringiensis and cloning of cryV-type genes from Bacillus thuringiensis subsp. kurstaki and Bacillus thuringiensis subsp. entomocidus. Appl Environ Microbiol 61:2402–2407
Theunis W, Aguda RM, Cruz WT, Decock C, Peferoen M, Lambert B, Bottrell DG, Gould FL, Litsinger JA, Cohen MB (1998) Bacillus thuringiensis isolates from the Philippines: habitat distribution, delta-endotoxin diversity, and toxicity to rice stem borers (Lepidoptera: Pyralidae). Bull Entomol Res 88:335–342
Tounsi S, Dammak M, Rebaî A, Jaoua S (2005) Response of larval Ephestia kuehniella (Lepidoptera: Pyralidae) to individual Bacillus thuringiensis kurstaki toxins and toxin mixtures. Biol Control 35:27–31
Travers RS, Martin PAW, Reichelderfer CF (1987) Selective process for efficient isolation of soil Bacillus species. App Environ Microbiol 53:1263–1266
Venables WN, Smith DM (2004) The R. development core team. An introduction to R. version 1.9.1. http://www..r-project.org/
Yang XW, Walker MJ, Hornitzky M, Chin J (2006) Development of a group-specific PCR combined with ARDRA for the identification of Bacillus species of environmental significance. J Microbiol Methods 64:107–119
Zouari N, Jaoua S (1999) The effect of complex carbon and nitrogen, salt, Tween-80 and acetate on delta-endotoxin production by a Bacillus thuringiensis subsp. kurstaki. J Ind Microbiol Biotechnol 23:497–502
Zouari N, Dhouib A, Ellouz R, Jaoua S (1998) Nutritional requirement of a strain of Bacillus thuringiensis subsp. Kurstaki and use of gruel hydrolysate, for the formulation of a new medium for delta-endotoxin production. Appl Biochem Biotechnol 69:41–52
Acknowledgments
This work was supported by grants from the “Ministry of Higher Education, Scientific Research and Technology” and the AUF “Agence Universitaire de la Francophonie”. We thank Dr. Patrick Schultz, Institute of Genetics and Molecular and Cellular Biology, for his help in electron microscopy experiments and Pr. Ahmed Rebai, Centre of Biotechnology of Sfax, for his help in statistical analysis.
Author information
Authors and Affiliations
Corresponding author
Additional information
Communicated by Erko Stackebrandt.
Rights and permissions
About this article
Cite this article
Saadaoui, I., Rouis, S. & Jaoua, S. A new Tunisian strain of Bacillus thuringiensis kurstaki having high insecticidal activity and δ-endotoxin yield. Arch Microbiol 191, 341–348 (2009). https://doi.org/10.1007/s00203-009-0458-y
Received:
Revised:
Accepted:
Published:
Issue Date:
DOI: https://doi.org/10.1007/s00203-009-0458-y