Abstract
Increasing concerns for global climate change has prompted search for a sustainable, efficient and renewable technology that can produce energy from wastewater. Microbial carbon capture cell (MCC) is an advancement of microbial fuel cell (MFC) in which algal biomass provides the oxygen for cathodic reduction in the cathodic chamber. Proof of concept of ability of MCC for organic matter removal from wastewater in anodic chamber along with simultaneous electricity generation and carbon sequestration in the cathodic chamber without the need of any external energy input has already been demonstrated. Algae is used in MCCs to supply oxygen for cathodic reduction and harvested algae, with or without oil extraction, can be fed as an organic substrate for bacteria in anodic chamber as well as it can act as methanogen inhibitor. Algal species used, its concentration in cathodic chamber, characteristics of wastewater used as feed in anodic chamber and catholyte composition, operating conditions and basic configuration of MCC affect performance of this device. This chapter describes the potential use of algal biomass in wastewater treatment and power generation, when incorporated in an MCC. Factors governing the performance of MCC and its possible applications are also discussed.
Access provided by Autonomous University of Puebla. Download chapter PDF
Similar content being viewed by others
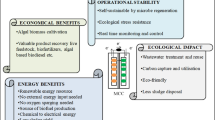
Keywords
- Bioelectricity
- Carbon sequestration
- Microbial carbon capture cell
- Microbial fuel cell
- Wastewater treatment
1 Introduction
Water quality has been degraded due to receiving partially treated or untreated wastewater from domestic and industrial sources as well as from non-point sources. In recent years, exponential population growth and accelerated industrial development has resulted in increased quantity of generation of wastewater with high concentrations of diverse nature of pollutants. Hence, maintaining water quality in the water bodies has become an utmost important issue that needs immediate attention.
On the other hand, depletion of fossil fuel reserves has prompted the need of developing sustainable energy generation technologies. One such innovative and renewable technology is microbial fuel cell (MFC), which can simultaneously treat organic matter from wastewater as well as generate energy in the form of electricity for onsite applications. These bio-electrochemical cells convert chemical energy from wastewater used as fuel into electricity. Several researches are being carried out with a goal to facilitate and accelerate the development of MFC, which uses microorganisms to transform the chemical energy present in organic compound into electricity (Logan et al. 2006).
Generally, MFC is composed of an anodic and cathodic chamber, which is separated by a proton exchange membrane (separator). In the anodic chamber, the exoelectrogenic microorganisms, also known as electrogens, act as biocatalysts and oxidize organic matter into electrons, protons and carbon dioxide. The electrons are transferred to the cathode via external electrical circuit, and protons are transferred into cathodic chamber through membrane. Oxygen, or any another chemical oxidant, combines with electrons and protons on cathode to favour oxygen reduction reaction to form water, or other reduced compounds if alternate chemical oxidants are used. By this process, the energy stored in the chemical bonds of organic matter present in wastewater can be converted to electricity, which could be used directly. Thus, an efficient but easily available electron acceptor and PEM are prerequisite for good performance of MFC (Pant et al. 2010). The cost of external mechanical aeration in cathodic chamber for supplying oxygen and the high cost of ion exchange membrane need to be minimized for field scale application of MFC for wastewater treatment and electricity generation.
High oxidation potential and clean reduction product (water) make oxygen as the most common electron acceptor to be used in MFC. On the other hand, supply of oxygen in the cathodic side is, however, energy intensive mainly due to energy cost associated with external mechanical aeration. Microbial carbon capture cells (MCCs) nullify this challenge by using the ability of algae to produce oxygen with an added advantage of using harvested algae as a feed stock for production of emerging biodiesel as a fuel source. MCCs with different configurations (Elmekawy et al. 2014), different electrodes (Wang et al. 2010), various substrates (Wu et al. 2014; Ganesh and Jambeck 2013) and numerous algal species (Cui et al. 2014; Saba et al. 2017) have been widely studied. Microalgae employed in cathodic chamber should have high photosynthetic productivity and higher lipid content, which can qualify it as a good feedstock for biodiesel production (Morita et al. 2000).
MCC is a mix of employing different processes in two different compartments and hence, the performance of MCC rely upon individual efficiency of these two distinct processes occurring in anodic and cathodic compartments, depending on operating conditions and design parameters. The interrelationship of these dependable variables basically influences the overall performance of MCC. The present chapter provides a detailed knowledge on MCC along with an idea about the prominent and relevant parameters that influence its performance and efficiency.
2 Microbial Carbon Capture Cell
Carbon capture can be achieved in MFC by modifying it to MCC, for providing an encouraging solution of utilizing the CO2 to synthesize the algal biomass in cathodic chamber, upon harvesting which can act as feedstock for biodiesel production, with simultaneous removal of organic matter from wastewater in anodic chamber and energy recovery in the form of electricity. The MCC consist of integration of MFC and algal species cultured in cathodic chamber for CO2 sequestration and O2 production to support cathodic reduction reaction (Fig. 1). The use of algal species in cathodic chamber generates oxygen during photosynthesis, making it available for cathodic reduction, thus reducing the cost of external aeration as required in aqueous cathode MFC.
In MCC, the CO2 generated during oxidative degradation of organic matter in the anodic chamber can be used for synthesis of the useful algal biomass with photosynthetic activity, attaining simultaneous electricity generation, CO2 sequestration, wastewater treatment and biomass production. Thus, this addition of anodic off gases, produced during oxidation of organic matter, into cathodic chamber for favouring algae growth in cathodic chamber demonstrates an effective way for reducing CO2 emission and in addition provides opportunity for simultaneous electricity generation without need of external aeration (Wang et al. 2010).
The overall biochemical reactions that occur at the anode and at the cathode of the MCC are as illustrated in Eq. (1) and Eqs. (2 and 3), respectively.
Anodic chamber:
Cathodic chamber (during exposure to light):
During light phase, algae carry out photosynthesis to produce oxygen and algal biomass, whereas during the dark phase, respiration occurs where the oxygen is consumed as per reaction explained in Eq. (4) (González et al. 2013).
Hence, there is a need to maintain the optimum light: dark period to get best possible performance from MCC.
3 Advancement in MCC
Research efforts on MCC mainly focused on the enhancement of performance and byproduct synthesis by optimizing the cathodic configuration and operating conditions, making favourable environment to support algal growth and enhancing cathodic reaction kinetics. Several aspects of MCC research have been discussed here.
3.1 Application of MCC
3.1.1 Wastewater Treatment
The organic matter present in the wastewater can be utilized as a carbon source for microbial communities in anodic chamber during oxidation, and, thus, wastewater can be effectively treated in MCC (Fig. 2). Pant et al. (2010) reviewed utilization of various substrates ranging from simple sugars (e.g. glucose, acetate) to complex wastewaters (e.g. starch processing wastewater, kitchen wastewater). The agricultural wastewater, domestic wastewater, effluent from food-processing industries, ligno-cellulosic waste, animal waste, waste activated sludge, etc. can be effectively treated in MFC, hence also in MCC, along with generation of bioelectricity. Hou et al. (2016) used Golenkinia sp. in cathodic chamber of MCC and achieved a power density of 6.3 W/m3 along with chemical oxygen demand (COD) removal of 44%. Some of the researchers have even used algal biomass as a substrate for anodic oxidation and produced higher power output (Rajesh et al. 2015). Further, integrating microalgae with MFC (i.e. MCC) will provide an added advantage of removing nutrients from wastewater streams, if provided at cathodic side, along with carbon capture (Neethu and Ghangrekar 2017).
3.1.2 Electricity Generation
Pandit et al. (2012) supplied CO2–air mixture to Anabaena sp. grown in cathodic chamber of MCC and generated a power density of 57.8 mW/m2. The electrical output from MCC varies with fluctuations in oxygen concentration synthesized by algal species. Jadhav et al. (2017) reported 1.5 fold higher power generation with use of Chlorella pyrenoidosa algal species over Anabaena ambigua sp., due to superiority in oxygen production rate of Chlorella. However, the electricity generated in MCC depends upon many physiological conditions that favour bacteria in the anodic chamber, which include pH of anolyte, electrode materials, design parameters as well as cathodic conditions such as algal concentration, CO2 concentration, etc.
3.1.3 Carbon Capture and Algal Biomass Production
Large quantity of CO2, emitted from various conventional wastewater treatment methods for organic pollutant degradation, is added to the environment annually (Campos et al. 2016). Capturing and supplying this extent of CO2 to the cathodic chamber of MCC will result in high algal biomass production by making the process sustainable with additional benefits (Kokabian et al. 2013). Additionally, microalgae have been recognized as one of the most productive biological systems for generating biomass and capturing carbon while treating the wastewater (Fig. 2). Considering properties of each algal species, the particular class of Chlorella sp. have high photosynthetic rate that favours high growth kinetics and results in higher biomass production. During MCC operation of 25 days, an algal biomass production of 66.4 mg/(L.day) was reported for Chlorella sp. and over 50.4 mg/(L.day) for Anabaena sp. grown in cathodic chamber due to variations in cell structure and growth kinetics (Jadhav et al. 2017). However, the carbon capture rate and biomass generation yield for individual algae depends upon the growth kinetics, nutrient availability for growth, operating conditions, light intensity and other environmental parameters.
3.1.4 Other Applications
Along with above discussed applications, the electricity generated from MCC can be capable to power the different electronic biosensors and electronic appliances. Additionally, algae in cathodic chamber are capable to remove nutrients from the anodic effluent and thus provide the polishing treatment before disposal of treated effluent (Fig. 2). The algal biomass harvested during photosynthesis can be further used as feedstock for synthesizing byproducts such as biodiesel, bioethanol, biohydrogen, methane, etc. (Wang et al. 2015). Also, while treating sewage, first it can be treated in anodic chamber for organic matter removal and then in cathodic chamber for removal of nutrient, and in this way higher pathogen destruction in cathodic chamber is also expected, producing superior quality treated water for desired onsite reuse.
3.2 Photosynthetic Algal Microbial Fuel Cell
Algae have been used commonly in MFCs to produce oxygen in the cathodic chamber so as to have oxygen reduction at cathode and harvested algal biomass, with or without oil extraction, can be utilized as a substrate for bacteria. However, sufficient electric current can also be generated at anode, where cytochromes help indirect shuttling of electrons generated in photosystem II of the algal cells and can be called as photosynthetic algal microbial fuel cell (PAMFC) (Shukla and Kumar 2018). He et al. (2014) reported a COD removal up to 92% with power density of 2.5 W/m3 in PAMFC having C. vulgaris in cathodic chamber. The immobilization of microalgal cells on polymeric or biopolymeric matrices can help in separation of algal cells together with enhancement of performance of MCC. Immobilization of microalgae provides additional advantage of increased cell density, resistance to toxic matter and stable operation with high metabolic activities over time.
3.3 Prospective of Algae
3.3.1 Algae as Substrate
Biomass is a good choice of feed stock to convert it to electrical energy, and algae are the most easily available source of biomass with high yield per unit area of land. The algal dry mass, considered as major pollutant vector in the streams, can be used as a potential substrate for electricity generation in anodic chamber of MCC (Cui et al. 2014). The algal biomass harvested during cathodic photosynthesis can be pretreated with heat treatment, enzymatic treatment or chemical treatment so that treated (or even untreated or live) algal mass can be utilized as a carbon source for anodic oxidation to produce the electrons (Shukla and Kumar 2018).
3.3.2 Algae as Biocathode
Since algae are photosynthetic microorganisms, the availability of light as well as the electron-donating anodic process may have significant effects on the performance of the biocathode. The phototrophic microorganisms can serve as biocatholytes in MFCs because the oxygen produced is an electron acceptor for the harvested electrons from the anodic chamber. Researchers used photosynthetic biocathode in sediment type MCC and achieved effective wastewater treatment (Commault et al. 2014; Neethu and Ghangrekar 2017). Previously, researchers also confirmed the advantageous use of algae as a viable biocathode in microbial desalination cells to supply electron acceptors in an sustainable manner (Kokabian and Gude 2015).
3.3.3 Algae as Inhibitor of Methanogens
Considering the varieties of microbial populations present in the mixed anaerobic sludge inoculum used in anodic chamber, major substrate is consumed by methanogenic consortia for methane production and other non-electrogenic reactions. To reduce the substrate consumption by non-electrogenic bacteria and recover maximum coulombs from the substrate, researchers have used algal powder to suppress the growth of methanogens and also to serve as a substrate for anodic oxidation (Rajesh et al. 2015, 2014). Hexadecatrienoic acid, a long-chain saturated fatty acid, present in the marine algae Chaetoceros was found to inhibit the growth of methanogenic archaea by the process of adsorption as well as disruption of cell membranes and achieved the Coulombic efficiency (CE) of 45.18% with a power density of 21.43 W/m3 (Rajesh et al. 2015).
3.3.4 Algae for Wastewater Treatment
Along with carbon capture, microalgae can assist in effective removal of nutrients present in wastewater by utilizing these elements during their cell metabolism (Elmekawy et al. 2014). Electro-migration along with diffusion of ions from anodic to cathodic chamber concurred for recovery of nutrients from the wastewater (Colombo et al. 2017). Recently, Huang et al. (2017b) reported maximum PO43−-P removal up to 37.2% using C. vulgaris biocathode in MFC. Nitrogen is utilized as nutrient source for algal cultivation and can be effectively treated in MCC at loading of 2 g/L of nitrate (Neethu et al. 2018). However, additional photo-bioreactor coupled with MFC is reported to be capable of removing about 92% phosphorous and 99% NH4+-N. Moreover, Kokabian and Gude (2015) proposed coupling of algae in cathodic chamber of microbial desalination cell and achieved complete salt removal in such system. Single-chambered air cathode MFC was capable of removing COD, colour and heavy metals (Zn-98%; Cr-80%) from wastewater (Logroño et al. 2017).
3.3.5 Algae as a Carbon Source for Electrode Material
Harmful algal blooms, including blue-green algae, can act as a promising electrode material for sodium-ion batteries and can be used for fuel cell applications. The algae carbonization helps to develop low-cost green electrode material for high-capacity batteries and also contribute to solve the issue of harmful algal blooms (Meng et al. 2015). Also, nanoporous carbon having large specific surface area can be synthesized from microalgae as a promising composite electrode material (Zhou et al. 2012).
3.3.6 Microalgal Biorefinery
Microalgae contains high concentration of proteins, lipids and carbohydrates, and its biomass after harvesting can be utilized as a potential feedstock for biodiesel production, lipid extraction and other applications. In microalgae biorefinery applications, CO2-neutral MFC was developed for producing feedstock for bioethanol production, algae oil extraction and bioelectricity generation simultaneously during treatment of fermented beer yeast in the anodic chamber (Powell and Hill 2009). However, some algal species requires pretreatment to release carbohydrates stored in the cells. In addition, photobiological and fermentative ways of biohydrogen production from algal biomass employing S. obliquus and Chlamydomonas reinhardtii in cathodic chamber of MCC are well established.
4 Factors Governing the Performance of MCC
4.1 Algal Biocathode
Oxygen is one of the most widely recognized electron acceptors utilized within the cathodic chamber of MFC owing to its high oxidation potential (0.8 V vs. SHE), and certainty it produces a clean end product, i.e. water, after reduction (Ucar et al. 2017). In any case, most investigations demonstrated that supply of oxygen to the cathodic chamber consumes energy. Microalgae may provide a viable alternative to cathodic oxygen supply; however, its efficiency as an eminent biocathode depends on various factors which are discussed briefly here. The oxygen that is given away during photosynthesis originates from water and not from the part of CO2. The light reaction responsible for oxygen production occurs at the thylakoid membranes of the cell chloroplast, thus rate of oxygen evolution is believed to be dependent mainly on cell type and concentration, light intensity and operating conditions (Perrine et al. 2012).
4.1.1 Algal Species, Type and its Concentration
Performance of MCC is reliant on algal species as the photosynthesis rate and cell multiplying time are diverse for different types of microalgae communities (Sun et al. 2016). An investigation by Jadhav et al. (Jadhav et al. 2017) showed the dominance of Chlorella over Anabaena for capturing CO2 and generation of photosynthetic oxygen that encouraged the cathodic reduction. The Chlorella sp. is the most preferred biocathode on account of their resistance for high level of CO2, higher tolerance to municipal wastewater and reasonable lipid content. A study utilizing diverse types of Chlorella in cathodic chamber showed that a superior performing MCC was acquired with C. vulgaris as biocathode to demonstrate higher biomass yield and CO2 fixation rate (Hu et al. 2015).
Gautam (2016) reported that biocathode with mixed algae collected from natural pond performed better over pure culture of C. pyrenoidosa biocathode in MCC under natural sunlight conditions and it can be suitable option for practical application of MCC at large scale. In this way the power yield is, by implication, dependent on the biomass concentration, which in turn relies upon algal species used and cell doubling time. Equally important is the cell concentration; lower cell concentration infers less oxygen (electron acceptor) availability, thus resulting in reduction in performance of MCC. Power can be enhanced with increment in concentration of algal biomass up to a certain concentration; however, beyond certain concentration of algae, the efficiency decreases due to self-shading of algae where cells close to the surface utilize a portion of the light and shade those more deep in the water (Ugwu and Aoyagi 2008). Another critical unfavourable impact of higher cell concentration is the metabolic loss because of formation of excess metabolites. Algae used in MCC serve as feedstock for biodiesel production as well as sequesters the anodic off gas introduced into the cathodic chamber. Hence, while choosing the algal species, it is crucial to choose species that have high photosynthetic efficiency to capture CO2 and also that can yield lipid having proper unsaturated fats for biodiesel synthesis.
4.1.2 Light, CO2 Supply and Oxygen Concentration
Different environmental parameters influence algal growth kinetics either directly or in a roundabout way, these include light/irradiance/temperature, CO2, pH, blending/aeration, salinity, etc. Keeping in mind one of the end goals, i.e. to improve the microalgal growth in cathodic chamber of MCC, the light prerequisite is a standout among the most vital parameters; hence, proper intensity, duration and wavelength of light should be provided with extreme care. Extreme intensity may prompt photo oxidation leading to growth prevention, while reduced light intensity will lead to growth restrictions. There have been a few advancement in the utilization of light by using changed light sources, such as texturized optical filaments and LEDs with particular wavelengths and proper lens to collimate light beam (Carvalho et al. 2011). The part of incident light that isn’t utilized gets changed over into heat energy; consequently, utilization of red and blue light is ideal to keep up the survivable temperature for algal cultivation (Michael et al. 2015). Thus, the utilization of internally illuminated LED can bring out different favourable circumstances of less heat energy scattering along with avoidance of self-shading. Low temperatures increase the solubility of CO2, and this indeed promotes the high growth rate and yield of microalgae.
Equally important is the presence of carbon source, especially CO2. Some green algae are accounted for to effortlessly grown at high CO2 concentration, and Chlorella are one of the known species that are used for carbon sequestration having high photosynthetic efficiency to convert CO2 to O2 (Singh and Singh 2014). Enhancement in power generation, quantity of biomass and lipids with increase in CO2 concentration were reported earlier by many authors (Wang et al. 2010; Sato et al. 2003; Andersen and Andersen 2006; Tang et al. 2011). Optimum concentration of CO2 varies with species as evident in the case of C. vulgaris with a carbon fixation rate of 6.17 mg/L.h (Bhola et al. 2011) unlike Scenedesmus species having the optimal CO2 consumption rate of 59.19 mg/L.h (Ho et al. 2012). When CO2 is consumed in the presence of light due to cell metabolic reactions, O2 is liberated, which acts as electron acceptor in MCC. One of the significant limitations of algal growth in a photo-bioreactor is that high oxygen concentration suppresses the growth, which is not an issue in MCC as oxygen released constantly get reduced by means of oxygen reduction reaction (ORR) for cathodic reduction. In MCC, a voltage of 706 mV was observed with a DO concentration of 6.6 mg/L in cathodic side, hence making algae as a suitable candidate for production of O2 that is necessary for stable performance of MCC (Kang et al. 2003).
4.1.3 Nitrate Concentration
Different microalgal species will respond differently to concentration of nutrients provided depending on their quota flexibility. Microalgae expel nutrients from wastewater essentially by utilizing it for algal metabolism (Aslan and Kapdan 2006). Nitrogen has a key impact in deciding the productivity of microalgae as far as biomass and lipid production is concerned. Neethu et al. (2018) reported that power generation was increased with increase in nitrate concentration from 0.5 to 2.0 g/L and further increase in nitrogen resulted into decrease in power output of MCC. Also, Converti et al. showed that decrease in concentration of nitrogen in the medium can expand the lipid portions of biomass dry weight (Converti et al. 2009). Hence, it is important to have an optimum concentration of nitrogen at which biomass and lipid content can be boosted. For this situation, the domestic wastewater, which contains moderately less inorganic nutrients, can permit appropriate development of microalgae alongside the accumulation of lipid content in algal cells.
4.2 Operating Conditions and Anodic Constraints
Performance of MCC is highly determined by the operating condition as discussed earlier. Thus, if the condition is unfavourable to the exoelectrogenic bacteria (on anode) and algal community (in cathodic chamber), the overall performance of MCC will be adversely affected. For optimum COD removal and power production, operating conditions such as organic loading rate (OLR), hydraulic retention time (HRT), inoculums, substrate, anodic environment and anolyte pH ought to be rightly chosen. The OLR mainly depends on the substrate concentration (i.e. COD loading) and flow rate, which is again dependent on HRT. Substrate fed in MFC can range from complex molecules of starch to simple acetate (Pant et al. 2010). Complex substrates have to be broken down to simple organic molecules before being used as carbon source in MFC for easy metabolism by bacteria (Pant et al. 2010). In a study, when acetate was compared with butyrate, propionate and glucose as substrate in MFC, the acetate-fed MFC gave the highest CE (Chae et al. 2009). Similar results proving the higher efficiency of MFC by usage of simple compounds were reported by Liu et al. (2009), which compared the efficiency of acetate-induced consortia and protein-rich wastewater as substrate. Operation mode can be either batch mode or continuous mode, where the later one is preferred in practical application for continuous generation of electricity. Also, the HRT to be provided depends mainly on the complexity or degradability of the substrate, the influence of which on performance of MFC being reported in several literature (Sharma and Li 2010; Akman et al. 2013; Rahimnejad et al. 2011). Hence an optimum HRT should be fixed, which gives the best result in the prevailing environmental, bacterial as well as other operating conditions.
In addition to the previously mentioned parameters, electrolyte pH is likewise as vital as it governs the performance of MFC. Majority of researches arrived at a conclusion that the consortia best performs in alkaline condition (Zhuang et al. 2010; Puig et al. 2010; Behera and Ghangrekar 2009). The reason for this conclusion is differently addressed in various studies. Certain work demonstrated the higher internal resistance at lower pH (Behera and Ghangrekar 2009), whereas Yuan et al. focused on the effect of anolyte pH on electrocatalytic activity of anodic biofilms (Yuan et al. 2011); alongside Zhuang et al. explained this by more negative anodic potential as a result of alkaline pH (Zhuang et al. 2010).
4.3 Design Parameters
Proper design of MCC can lower the overall internal resistance and improve efficiency of organic matter oxidation and TDS removal. Design parameters that affect the performance include the electrode spacing, electrode material, membrane thickness and area, mixing, size of chambers and reactor layout. The reactor configuration is a critical parameter that influences the power generation and algal production in MCC (Table 1). Algae are generally cultivated in photo-bioreactor (PBR), and subsequently while incorporating algae in MFC system, it can take two configurations – the PBR externally connected to the MFC and the PBR incorporated inside the MFC components (Fig. 3).
The first configuration can be a photo-reactor bottle connected using peristaltic pump to the MFCs, where complete recirculation occurs in a closed-loop system (Gajda et al. 2015; Jiang et al. 2013) or PBR kept separately without PEM wherein CO2 generated in anodic chamber is directly released into the photo-bioreactor (Powell et al. 2009). In case of later, among the diverse reactor designs proposed by researchers, the generally adopted design is dual-chambered MCC.
In a two-chambered MCC, anolyte (anodic chamber) and catholyte (cathodic chamber) are separated by a membrane (proton exchange membrane), and electrodes are linked by an external circuit. In this type of MCC, the CO2 produced in anodic chamber is generally transferred to the cathodic chamber through a vent made at top of each chamber (Khandelwal et al. 2018). Apart from dual-chambered system, certain studies were carried out using single-chambered MCC, where the electrodes were placed in a single chamber without separators and algal bacterial symbiosis was observed in such MCC (Fu et al. 2010). Aside from this, an airlift MCC system, established by Hu et al. (2015), simultaneously achieved the high level of carbon sequestration along with wastewater remediation.
Design of algal chamber of MCC is one of the major technical aspects that should be given importance for the production of microalgal biomass in cathodic chamber. In contrast to the usual MFCs, there must be a transparent surface keeping in mind the end goal to guarantee the light is received by the algal cells in cathodic chamber of MCC. For the most effective utilization of incident light, several studies have been carried out in terms of reactor design and its architecture. While designing the cathodic part of MCC, no light should be lost, and no dark area ought to happen in which algae don’t grow. So light capturing, channelling and scattering play an important role while considering the design of MCC. Scientists have explored the utilization of Fresnel focal points and light guides to focus, carry and deliver direct light into the algae suspension (Zijffers et al. 2008). Also, few studies were conducted to enhance the horizontal dispersion to an expansive stretch out by roughening the surface of the illuminating surface of the distributor (Csögör et al. 1999). Hence, while planning the lighting arrangement to MCC, importance should be given to the following: (i) enough light is available as required for the growth of species used, (ii) light intensity that can be adjusted accordingly, (iii) light wavelength (blue, red) that can be shifted to support the algal growth, (iv) light frequency should be variable, which can match the prevailing condition of region, (v) uniform distribution of light throughout the media and (vi) proper mixing can help in uniform distribution of incident light.
Biocompatibility of carbon-based material such as graphite rod, carbon felt, carbon cloth, etc. makes it the most suitable material to be used as electrodes in MCC (Table 1). Surface area and its roughness are two major factors that determine the adhesion of bacterial or algal biofilm on electrode surface. Compared to other electrode materials, graphite felt provide the desired surface area and texture required for uniform biofilm formation and colonization of bacteria. An enriched biofilm formation on anode surface will help in easy transfer of electrons to anode. Another major design factor that determines the performance of an MCC is the spacing between anode and cathode. Though utilization of appropriate material can diminish the activation losses (Mustakeem 2015; Zhou et al. 2011), bringing the anode and cathode closer can bring down the ohmic losses (Doherty et al. 2015). The power generation decreases with an increasing electrode spacing; whereas, keeping the electrodes too close can quicken substrate and oxygen diffusion, bringing about fast biofouling of the cathodes (Tartakovsky and Guiot 2006). To overcome these unfavourable impacts on performance of MCC, there should be an ideal separation between the electrodes. Electrode spacing could be related with different factors in controlling efficiency of MFC. Previous studies showed the effect of external resistance (Ghangrekar and Shinde 2007), where a maximum power density was observed at lower spacing of 20 cm between the electrodes, apart from this certain studies proved that the control over electrode spacing for improving power generation is dependent on substrate concentration (Lee and Huang 2013). Ahn et al. have tried different electrode configurations to optimize the performance of a multi-electrode MFC; a better power and coulombic efficiency was attained by separator electrode assembly configuration, whereas better wastewater treatment efficiency was achieved in configuration with closely spaced electrodes (Ahn et al. 2014).
Maintaining the anaerobicity of anodic chamber is a prerequisite for the growth of exoelectrogenic bacteria in anodic chamber of MCC. Hence, it is necessary to set apart the anodic chamber from the cathodic chamber rich in oxygen, and here separator plays a major role in design of MCC. An ideal separator should have higher proton conductivity, ion transport number, ion exchange capacity, water absorption along with minimal oxygen diffusion, resistance, acetate crossover and biodegradability (Tanaka 2015). Among the different cation exchange membranes commonly used, Nafion is most popularly used membrane (Huang et al. 2017a); however, bipolar membranes (Kim et al. 2017), chitosan-graphene oxide mixed-matrix membrane (Holder et al. 2017), glass wool (Venkata Mohan et al. 2008), SPEEK membranes (Ghasemi et al. 2016), ceramic membranes (Daud et al. 2018) and clayware membranes (Ghadge et al. 2015) were also used. The mostly used membrane separator in MFC is the Nafion membrane; however, it has few limitations, for example, oxygen diffusion, cation accumulation, substrate loss, durability and high cost. These confinements have prompted tremendous attempts in the advancement of a suitable material that can viably fill in as a low-cost PEM, and the researches are still going on in search of suitable replacement.
4.4 Other Factors
Along with major factors, other parameters such as mixing conditions, immobilization of biomass, etc. affect the performance of MCC in terms of electricity generation and wastewater treatment. Turbulence (mixing) affects the growth positively by increasing rate of mass transfer between nutrients and algal cell; it also helps in removal of metabolites (e.g. oxygen) from the growth media. Similar studies also suggested immobilization of microalgae on glass beads or polymeric or biopolymeric matrices are capable of producing high algal cell concentration, resistance to hazardous matter, stable and flexible operation and longer period of operation with stable voltage due to longer logarithmic growth phase (Jin et al. 2011; Bashan and De-Bashan 2010). The rate of cathodic reactions was enhanced by concentration of oxygen as terminal electron acceptor, and hence growth kinetics of algal culture under given operating conditions is important to overcome the cathodic limitations.
5 Bottlenecks and Perspectives
Even though remarkable progress is evident in the field of MCC research, there are still certain challenges that need to be overthrown in order to commercialize this technology. Integrating algae in MFC will make MFC a complex system, whose performance will depend on several factors most of which have been already discussed in this chapter. Since in MCC the oxygen produced by the microalgae plays a major role, increasing the photosynthetic efficiency of algal species is of utmost importance. As discussed earlier, different microalgae strains respond differently to different growth conditions provided, and hence it is difficult to optimize these conditions to a specific species. Also, in MCC the algae relies upon the CO2 received from the anodic chamber; hence, studies need to be done to quantify the flow of anodic off gas towards the algal chamber, and further anodic oxidation will be limited by the O2 released by photosynthesis; hence, its role is important. MCC developed must be able to treat wastewater having different strengths and compositions; handle variations in pH, temperature, etc.; and operate without any adverse impact to the environment. Modelling studies and optimization of various factors affecting the performance of MCC are of great importance. For enhancing power generation, development/synthesis of low-cost cathode catalyst that is not toxic to algae is yet to be investigated.
Design parameters including the materials used and MCC configurations are equally important in increasing the efficiency of MCC. Configuration of MCC should be such that it gives no or minimal loss of CO2 while moving from anodic chamber to cathodic chamber. Along with transport of CO2, transport of protons from anodic chamber to cathodic chamber is equally important to complete the redox reactions. A low-cost membrane separator with minimal oxygen and acetate diffusion along with high proton conduction is prerequisite for efficient performance of MCC, which is yet to be synthesized. Similarly, the voltage generated has a positive correlation with the dissolved oxygen concentration of catholyte (Neethu et al. 2018), which fluctuates with the day and night cycle. This can come out as a great challenge faced by MCC, when thought to be operated under natural sunlight condition, which needs an immediate solution. Considering the complication of MFC system alone, coupling or integrating algal system to MFC makes overall process complex for commercialization. The high capital cost for fabricating MCC, considering all dependable components and their lower efficiencies, is also by far the major factor contributing to the limited commercialization of MCC technology. In order to compete realistically with other prevailing feedstock yield for biodiesel production and power generation technologies, as well as offering wastewater remediation, MCC should turn out to be more powerful from the viewpoint of both effectiveness and cost.
6 Summary
Application of MFC in wastewater treatment and bioelectricity generation is a well-known concept in present scenario; however, utilization of algae for oxygen supply, biomass production and other product synthesis along with providing polishing treatment to wastewater for removing nutrients is a major breakthrough in BES research domain. The performance of the system as a whole depends on the electrochemical reactions that occur between substrate oxidation to the final electron acceptor. Factors including algal growth kinetics, density, light intensity, CO2 supply and other operating conditions govern the performance of MCC. Proper selection of these parameters and finding optimum condition for these for the algal species being cultivated in cathodic chamber of MCC can take forward this technology to an advanced level for its real-life applications. Moreover, presence of algae in cathodic chamber of MCC, apart from providing oxygen for cathodic reduction, also can serve as a substrate for anodic oxidation, a methanogen inhibitor to enhance the CE, harvested algal biomass for biodiesel production and other byproduct recovery, which can make MCC a cost-effective and sustainable solution for wastewater treatment as compared to conventional wastewater treatment methods.
References
Ahn Y, Hatzell MC, Zhang F, Logan BE (2014) Different electrode configurations to optimize performance of multi-electrode microbial fuel cells for generating power or treating domestic wastewater. J Power Sources 249:440–445. https://doi.org/10.1016/j.jpowsour.2013.10.081
Akman D, Cirik K, Ozdemir S, Ozkaya B, Cinar O (2013) Bioelectricity generation in continuously-fed microbial fuel cell: effects of anode electrode material and hydraulic retention time. Bioresour Technol 149:459–464. https://doi.org/10.1016/j.biortech.2013.09.102
Andersen T, Andersen F (2006) Effects of CO2 concentration on growth of filamentous algae and Littorella Uniflora in a Danish Softwater Lake. Aquat Bot 84(3):267–271. https://doi.org/10.1016/j.aquabot.2005.09.009
Angelaalincy M, Senthilkumar N, Karpagam R, Kumar GG, Ashokkumar B, Varalakshmi P (2017) Enhanced extracellular polysaccharide production and self-sustainable electricity generation for PAMFCs by Scenedesmus Sp. SB1. ACS Omega 2(7):3754–3765. https://doi.org/10.1021/acsomega.7b00326
Aslan S, Kapdan IK (2006) Batch kinetics of nitrogen and phosphorus removal from synthetic wastewater by algae. Ecol Eng 28(1):64–70. https://doi.org/10.1016/j.ecoleng.2006.04.003
Bashan Y, De-Bashan LE (2010) Immobilized microalgae for removing pollutants: review of practical aspects. Bioresour Technol 101:1611–1627
Behera M, Ghangrekar MM (2009) Performance of microbial fuel cell in response to change in sludge loading rate at different anodic feed pH. Bioresour Technol 100(21):5114–5121. https://doi.org/10.1016/j.biortech.2009.05.020
Bhola V, Desikan R, Santosh SK, Subburamu K, Sanniyasi E, Bux F (2011) Effects of parameters affecting biomass yield and thermal behaviour of chlorella vulgaris. J Biosci Bioeng 111(3):377–382. https://doi.org/10.1016/j.jbiosc.2010.11.006
Cai PJ, Xiao X, He YR, Li WW, Zang GL, Sheng GP, Hon-Wah Lam M, Yu L, Yu HQ (2013) Reactive oxygen species (ROS) generated by cyanobacteria act as an electron acceptor in the biocathode of a bio-electrochemical system. Biosens Bioelectron 39(1):306–310. https://doi.org/10.1016/j.bios.2012.06.058
Campos JL, Valenzuela-Heredia D, Pedrouso A, Val Del Río A, Belmonte M, Mosquera-Corral A (2016) Greenhouse gases emissions from wastewater treatment plants: minimization, treatment, and prevention. J Chem 2016:1. https://doi.org/10.1155/2016/3796352
Carvalho AP, Silva SO, Baptista JM, Malcata FX (2011) Light requirements in microalgal photobioreactors: an overview of biophotonic aspects. Appl Microbiol Biotechnol 89(5):1275–1288. https://doi.org/10.1007/s00253-010-30478
Chae KJ, Choi MJ, Lee JW, Kim KY, Kim IS (2009) Effect of different substrates on the performance, bacterial diversity, and bacterial viability in microbial fuel cells. Bioresour Technol 100(14):3518–3525. https://doi.org/10.1016/j.biortech.2009.02.065
Colombo A, Marzorati S, Lucchini G, Cristiani P, Pant D, Schievano A (2017) Assisting cultivation of photosynthetic microorganisms by microbial fuel cells to enhance nutrients recovery from wastewater. Bioresour Technol 237:240–248. https://doi.org/10.1016/j.biortech.2017.03.038
Commault AS, Lear G, Novis P, Weld RJ (2014) Photosynthetic biocathode enhances the power output of a sediment-type microbial fuel cell. N Z J Bot 52(1):48–59. https://doi.org/10.1080/0028825X.2013.870217
Converti A, Casazza AA, Ortiz EY, Perego P, Del Borghi M (2009) Effect of temperature and nitrogen concentration on the growth and lipid content of Nannochloropsis Oculata and chlorella vulgaris for biodiesel production. Chem Eng Process Process Intensif 48(6):1146–1151. https://doi.org/10.1016/j.cep.2009.03.006
Csögör Z, Herrenbauer M, Perner I, Schmidt K, Posten C (1999) Design of a photo-bioreactor for modelling purposes. Chem Eng Process Process Intensif 38(4–6):517–523. https://doi.org/10.1016/S0255-2701(99)00048-3
Cui Y, Rashid N, Hu N, Rehman MSU, In Han J (2014) Electricity generation and microalgae cultivation in microbial fuel cell using microalgae-enriched anode and bio-cathode. Energy Convers Manag 79:674–680. https://doi.org/10.1016/j.enconman.2013.12.032
Daud SM, Daud WRW, Kim BH, Somalu MR, Bakar MHA, Muchtar A, Jahim JM, Lim SS, Chang IS (2018) Comparison of performance and ionic concentration gradient of two-chamber microbial fuel cell using ceramic membrane (CM) and cation exchange membrane (CEM) as separators. Electrochim Acta 259:365–376. https://doi.org/10.1016/j.electacta.2017.10.118
Doherty L, Zhao X, Zhao Y, Wang W (2015) The effects of electrode spacing and flow direction on the performance of microbial fuel cell-constructed wetland. Ecol Eng 79:8–14. https://doi.org/10.1016/j.ecoleng.2015.03.004
Elmekawy A, Hegab HM, Vanbroekhoven K, Pant D (2014) Techno-productive potential of photosynthetic microbial fuel cells through different configurations. Renew Sust Energ Rev 39:617–627. https://doi.org/10.1016/j.rser.2014.07.116
Fu CC, Hung TC, Wen Teng W, Wen TC, Chia Hung S (2010) Current and voltage responses in instant photosynthetic microbial cells with spirulina platensis. Biochem Eng J 52(2–3):175–180. https://doi.org/10.1016/j.bej.2010.08.004. Elsevier B.V.
Gajda I, Greenman J, Melhuish C, Ieropoulos I (2015) Self-sustainable electricity production from algae grown in a microbial fuel cell system. Biomass Bioenergy 82:1–7. https://doi.org/10.1016/j.biombioe.2015.05.017. Elsevier Ltd
Ganesh K, Jambeck JR (2013) Treatment of landfill leachate using microbial fuel cells: alternative anodes and semi-continuous operation. Bioresour Technol 139:383–387. https://doi.org/10.1016/j.biortech.2013.04.013
Gautam PK (2016) Study of effect of different operating parameters on performance of low cost clayware microbial carbon capture cell, M. Tech. Dissertation, IIT Kharagpur, West Bengal, India
Ghadge AN, Jadhav DA, Pradhan H, Ghangrekar MM (2015) Enhancing waste activated sludge digestion and power production using hypochlorite as catholyte in clayware microbial fuel cell. Bioresour Technol 182:225–231. https://doi.org/10.1016/j.biortech.2015.02.004. Elsevier Ltd
Ghangrekar MM, Shinde VB (2007) Performance of membrane-less microbial fuel cell treating wastewater and effect of electrode distance and area on electricity production. Bioresour Technol 98(15):2879–2885. https://doi.org/10.1016/j.biortech.2006.09.050
Ghasemi M, Wan Daud WR, Alam J, Jafari Y, Sedighi M, Aljlil SA, Ilbeygi H (2016) Sulfonated poly ether ether ketone with different degree of sulphonation in microbial fuel cell: application study and economical analysis. Int J Hydrog Energy 41(8):4862–4871. https://doi.org/10.1016/j.ijhydene.2015.10.029
González A, Cañizares P, Rodrigo MA, Fernández FJ, Lobato J (2013) Microbial fuel cell with an algae-assisted cathode: a preliminary assessment. J Power Sources 242:638–645. https://doi.org/10.1016/j.jpowsour.2013.05.110. Elsevier B.V
He H, Zhou M, Yang J, Hu Y, Zhao Y (2014) Simultaneous wastewater treatment, electricity generation and biomass production by an immobilized photosynthetic algal microbial fuel cell. Bioprocess Biosyst Eng 37(5):873–880. https://doi.org/10.1007/s00449-013-1058-4
Ho SH, Chen CY, Chang JS (2012) Effect of light intensity and nitrogen starvation on CO2 fixation and lipid/carbohydrate production of an indigenous microalga Scenedesmus obliquus CNW-N. Bioresour Technol 113:244–252. https://doi.org/10.1016/j.biortech.2011.11.133
Holder SL, Lee CH, Popuri SR (2017) Simultaneous wastewater treatment and bioelectricity production in microbial fuel cells using cross-linked chitosan-graphene oxide mixed-matrix membranes. Environ Sci Pollut Res 24(15):13782–13796. https://doi.org/10.1007/s11356-017-8839-2
Hou Q, Nie C, Pei H, Hu W, Jiang L, Yang Z (2016) The effect of algae species on the bioelectricity and biodiesel generation through open-air cathode microbial fuel cell with kitchen waste anaerobically digested effluent as substrate. Bioresour Technol 218:902–908. https://doi.org/10.1016/j.biortech.2016.07.035
Hu X, Liu B, Zhou J, Jin R, Qiao S, Liu G (2015) CO2 fixation, lipid production, and power generation by a novel air-lift-type microbial carbon capture cell system. Environ Sci Technol 49(17):10710–10717. https://doi.org/10.1021/acs.est.5b02211
Huang D, Song BY, He YL, Ren Q, Yao S (2017a) Cations diffusion in Nafion117 membrane of microbial fuel cells. Electrochim Acta 245:654–663. https://doi.org/10.1016/j.electacta.2017.06.004
Huang Y, Huang Y, Liao Q, Fu Q, Xia A, Zhu X (2017b) Improving phosphorus removal efficiency and chlorella vulgaris growth in high-phosphate MFC wastewater by frequent addition of small amounts of nitrate. Int J Hydrog Energy 42(45):27749–27758. https://doi.org/10.1016/j.ijhydene.2017.05.069
Jadhav DA, Jain SC, Ghangrekar MM (2017) Simultaneous wastewater treatment, algal biomass production and electricity generation in clayware microbial carbon capture cells. Appl Biochem Biotechnol 183(3):1076–1092. https://doi.org/10.1007/s12010-017-2485-5
Jiang HM, Luo SJ, Shi XS, Dai M, Guo RB (2013) A system combining microbial fuel cell with Photobioreactor for continuous domestic wastewater treatment and bioelectricity generation. J Cent South Univ 20(2):488–494. https://doi.org/10.1007/s11771-013-1510-2
Jin J, Yang L, Chan SMN, Luan T, Li Y, Tam NFY (2011) Effect of nutrients on the biodegradation of tributyltin (TBT) by alginate immobilized microalga, Chlorella vulgaris, in natural river water. J Hazard Mater 185:1582–1586
Kang KH, Jang JK, Pham TH, Moon H, Chang IS, Kim BH (2003) A microbial fuel cell with improved cathode reaction as a low biochemical oxygen demand sensor. Biotechnol Lett 25(16):1357–1361. https://doi.org/10.1023/A:1024984521699
Khandelwal A, Vijay A, Dixit A, Chhabra M (2018) Microbial fuel cell powered by lipid extracted algae: a promising system for algal lipids and power generation. Bioresour Technol 247:520–527. https://doi.org/10.1016/j.biortech.2017.09.119
Kim C, Lee CR, Song YE, Heo J, Choi SM, Lim DH, Cho J, Park C, Jang M, Kim JR (2017) Hexavalent chromium as a cathodic electron acceptor in a bipolar membrane microbial fuel cell with the simultaneous treatment of electroplating wastewater. Chem Eng J 328:703–707. https://doi.org/10.1016/j.cej.2017.07.077
Kokabian B, Gude VG (2015) Sustainable photosynthetic biocathode in microbial desalination cells. Chem Eng J 262:958–965. https://doi.org/10.1016/j.cej.2014.10.048
Kokabian B, Gude VG, Kokabian B, Gadhamshetty V (2013) Beneficial bioelectrochemical systems for energy, water, and biomass production. J Microb Biochem Technol:1–14. https://doi.org/10.4172/1948-5948.S6-005
Lee C-Y, Huang Y-N (2013) The effects of electrode spacing on the performance of microbial fuel cells under different substrate concentrations. Water Sci Technol 68(9):2028–2034. https://doi.org/10.2166/wst.2013.446
Liu Z, Liu J, Zhang S, Su Z (2009) Study of operational performance and electrical response on mediator-less microbial fuel cells fed with carbon- and protein-rich substrates. Biochem Eng J 45(3):185–191
Liu T, Rao L, Yuan Y, Zhuang L (2015) Bioelectricity generation in a microbial fuel cell with a self-sustainable photocathode. Sci World J 2015:1. https://doi.org/10.1155/2015/864568
Logan BE, Hamelers B, Rozendal R, Schröder U, Keller J, Freguia S, Aelterman P, Verstraete W, Rabaey K (2006) Microbial fuel cells: methodology and technology. Environ Sci Technol 40(17):5181–5192. https://doi.org/10.1021/es0605016
Logroño W, Pérez M, Urquizo G, Kadier A, Echeverría M, Recalde C, Rákhely G (2017) Single chamber microbial fuel cell (SCMFC) with a cathodic microalgal biofilm: a preliminary assessment of the generation of bioelectricity and biodegradation of real dye textile wastewater. Chemosphere 176:378–388. https://doi.org/10.1016/j.chemosphere.2017.02.099
Ma J, Wang Z, Zhang J, Waite TD, Wu Z (2017) Cost-effective chlorella biomass production from dilute wastewater using a novel photosynthetic microbial fuel cell (PMFC). Water Res 108:356–364. https://doi.org/10.1016/j.watres.2016.11.016
Meng X, Savage PE, Deng D (2015) Trash to treasure: from harmful algal blooms to high-performance electrodes for sodium-ion batteries. Environ Sci Technol 49(20):12543–12550. https://doi.org/10.1021/acs.est.5b03882
Michael C, del Ninno M, Gross M, Wen Z (2015) Use of wavelength-selective optical light filters for enhanced microalgal growth in different algal cultivation systems. Bioresour Technol 179:473–482. https://doi.org/10.1016/j.biortech.2014.12.075
Mitra P, Hill GA (2012) Continuous microbial fuel cell using a photoautotrophic cathode and a fermentative anode. Can J Chem Eng 90(4):1006–1010. https://doi.org/10.1002/cjce.20605
Morita M, Watanabe Y, Saiki H (2000) High photosynthetic productivity of green microalga chlorella Sorokiniana. Appl Biochem Biotechnol 87:203–218. https://doi.org/10.1385/ABAB:87:3:203
Mustakeem (2015) Electrode materials for microbial fuel cells: nanomaterial approach. Mater Renewable Sustainable Energy 4. https://doi.org/10.1007/s40243-015-0063-8
Neethu B, Ghangrekar MM (2017) Electricity generation through a photo sediment microbial fuel cell using algae at the cathode. Water Sci Technol 76(12):3269–3277. https://doi.org/10.2166/wst.2017.485
Neethu B, Goutam PK, Jadhav DA, Ghangrekar MM (2018) Simultaneous nutrient removal, algal biomass production and electricity generation in microbial carbon capture cell, Int. conference on Sustainable Technologies for Intelligent Water Management, Roorkee
Pandit S, Nayak BK, Das D (2012) Microbial carbon capture cell using cyanobacteria for simultaneous power generation, carbon dioxide sequestration and wastewater treatment. Bioresour Technol 107:97–102. https://doi.org/10.1016/j.biortech.2011.12.067
Pant D, Van Bogaert G, Diels L, Vanbroekhoven K (2010) A review of the substrates used in microbial fuel cells (MFCs) for sustainable energy production. Bioresour Technol 101(6):1533–1543. Elsevier Ltd. https://doi.org/10.1016/j.biortech.2009.10.017
Perrine Z, Negi S, Sayre RT (2012) Optimization of photosynthetic light energy utilization by microalgae. Algal Res 1(2):134–142. https://doi.org/10.1016/j.algal.2012.07.002
Powell EE, Hill GA (2009) Economic assessment of an integrated bioethanol–biodiesel–microbial fuel cell facility utilizing yeast and photosynthetic algae. Chem Eng Res Des 87(9):1340–1348. https://doi.org/10.1016/j.cherd.2009.06.018
Powell EE, Mapiour ML, Evitts RW, Hill G a (2009) Growth kinetics of chlorella vulgaris and its use as a cathodic half cell. Bioresour Technol 100(1):269–274. https://doi.org/10.1016/j.biortech.2008.05.032
Puig S, Serra M, Coma M, Cabré M, Balaguer MD, Colprim J (2010) Effect of pH on nutrient dynamics and electricity production using microbial fuel cells. Bioresour Technol 101(24):9594–9599. https://doi.org/10.1016/j.biortech.2010.07.082
Rago L, Cristiani P, Villa F, Zecchin S, Colombo A, Cavalca L, Schievano A (2017) Influences of dissolved oxygen concentration on biocathodic microbial communities in microbial fuel cells. Bioelectrochemistry 116:39–51. https://doi.org/10.1016/j.bioelechem.2017.04.001
Rahimnejad M, Ghoreyshi AA, Najafpour G, Jafary T (2011) Power generation from organic substrate in batch and continuous flow microbial fuel cell operations. Appl Energy 88(11):3999–4004. https://doi.org/10.1016/j.apenergy.2011.04.017
Rajesh PP, Noori MT, Ghangrekar MM (2014) Controlling methanogenesis and improving power production of microbial fuel cell by lauric acid dosing. Water Sci Technol 70(8):1363–1369. https://doi.org/10.2166/wst.2014.386
Rajesh PP, Jadhav DA, Ghangrekar MM (2015) Improving performance of microbial fuel cell while controlling methanogenesis by Chaetoceros pretreatment of anodic inoculum. Bioresour Technol 180:66–71. https://doi.org/10.1016/j.biortech.2014.12.095
Saba B, Christy AD, Zhongtang Y, Co AC (2017) Sustainable power generation from bacterio-algal microbial fuel cells (MFCs): an overview. Renew Sust Energ Rev 73:75. https://doi.org/10.1016/j.rser.2017.01.115
Sato N, Tsuzuki M, Kawaguchi A (2003) Glycerolipid synthesis in Chlorella Kessleri 11 H - II. Effect of the CO2 concentration during growth. Biochim Biophys Acta Mol Cell Biol Lipids 1633(1):35–42. https://doi.org/10.1016/S1388-1981(03)00070-2
Sharma Y, Li B (2010) Optimizing energy harvest in wastewater treatment by combining anaerobic hydrogen producing biofermentor (HPB) and microbial fuel cell (MFC). Int J Hydrog Energy 35(8):3789–3797. https://doi.org/10.1016/j.ijhydene.2010.01.042
Shukla M, Kumar S (2018) Algal growth in photosynthetic algal microbial fuel cell and its subsequent utilization for biofuels. Renew Sust Energ Rev 82:402–414
Singh SP, Singh P (2014) Effect of CO2 concentration on algal growth: a review. Renew Sust Energ Rev 38:172–179. https://doi.org/10.1016/j.rser.2014.05.043
Sun Z, Liu J, Zhou ZG (2016) Algae for biofuels: an emerging feedstock. An emerging feedstock. In: Handbook of biofuels production: processes and technologies: second edition, pp 673–698. https://doi.org/10.1016/B978-0-08-100455-5.00022-9
Tanaka Y (2015) Ion exchange membranes. https://doi.org/10.1016/B978-0-444-63319-4.00012-2
Tang D, Han W, Li P, Miao X, Zhong J (2011) CO2 biofixation and fatty acid composition of Scenedesmus Obliquus and Chlorella Pyrenoidosa in response to different CO2 levels. Bioresour Technol 102(3):3071–3076. https://doi.org/10.1016/j.biortech.2010.10.047
Tartakovsky B, Guiot SR (2006) A comparison of air and hydrogen peroxide oxygenated microbial fuel cell reactors. Biotechnol Prog 22:241–246. https://doi.org/10.1021/bp050225j
Ucar D, Zhang Y, Angelidaki I (2017) An overview of electron acceptors in microbial fuel cells. Front Microbiol 8. https://doi.org/10.3389/fmicb.2017.00643
Ugwu CU, Aoyagi H (2008) Influence of shading inclined tubular Photobioreactor surfaces on biomass productivity of C. Sorokiniana. Photosynthetica 46(2):283–285. https://doi.org/10.1007/s11099-008-0049-1
Venkata Mohan S, Veer Raghavulu S, Sarma PN (2008) Biochemical evaluation of bioelectricity production process from anaerobic wastewater treatment in a single chambered microbial fuel cell (MFC) employing glass wool membrane. Biosens Bioelectron 23(9):1326–1332. https://doi.org/10.1016/j.bios.2007.11.016
Wang X, Feng Y, Liu J, Lee H, Li C, Li N, Ren N (2010) Sequestration of CO2 discharged from anode by algal cathode in microbial carbon capture cells (MCCs). Biosens Bioelectron 25(12):2639–2643. https://doi.org/10.1016/j.bios.2010.04.036
Wang H, Lu L, Liu D, Cui F, Wang P (2015) Characteristic changes in algal organic matter derived from Microcystis aeruginosa in microbial fuel cells. Bioresour Technol 195:25–30. https://doi.org/10.1016/j.biortech.2015.06.014
Wu Y-c, Wang Z-j, Zheng Y, Xiao Y, Yang Z-h, Zhao F (2014) Light intensity affects the performance of photo microbial fuel cells with Desmodesmus Sp. A8 as cathodic microorganism. Appl Energy 116:86–90. https://doi.org/10.1016/j.apenergy.2013.11.066
Xu C, Poon K, Choi MMF, Wang R (2015) Using live algae at the anode of a microbial fuel cell to generate electricity. Environ Sci Pollut Res 22:15621. https://doi.org/10.1007/s11356-015-4744-8
Yang Q, Lin Y, Liu L, Yang F (2017) A bio-electrochemical membrane system for more sustainable wastewater treatment with MnO2/PANI modified stainless steel cathode and photosynthetic provision of dissolved oxygen by algae. Water Sci Technol 76(7):1907–1914. https://doi.org/10.2166/wst.2017.301
Yuan Y, Zhao B, Zhou S, Zhong S, Zhuang L (2011) Electrocatalytic activity of anodic biofilm responses to pH changes in microbial fuel cells. Bioresour Technol 102(13):6887–6891. https://doi.org/10.1016/j.biortech.2011.04.008
Zheng W, Cai T, Huang M, Chen D (2017) Comparison of electrochemical performances and microbial community structures of two photosynthetic microbial fuel cells. J Biosci Bioeng 124(5):551–558. https://doi.org/10.1016/j.jbiosc.2017.05.013
Zhou M, Chi M, Luo J, He H, Jin T (2011) An overview of electrode materials in microbial fuel cells. J Power Sources 196:4427. https://doi.org/10.1016/j.jpowsour.2011.01.012
Zhou M, He H, Jin T, Wang H (2012) Power generation enhancement in novel microbial carbon capture cells with immobilized chlorella vulgaris. J Power Sources 214:216–219. https://doi.org/10.1016/j.jpowsour.2012.04.043. Elsevier Ltd
Zhuang L, Zhou S, Li Y, Yuan Y (2010) Enhanced performance of air-cathode two-chamber microbial fuel cells with high-pH anode and low-pH cathode. Bioresour Technol 101(10):3514–3519. https://doi.org/10.1016/j.biortech.2009.12.105
Zijffers JWF, Salim S, Janssen M, Tramper J, Wijffels RH (2008) Capturing sunlight into a photobioreactor: ray tracing simulations of the propagation of light from capture to distribution into the reactor. Chem Eng J 145(2):316–327. https://doi.org/10.1016/j.cej.2008.08.011
Acknowledgement
Grants received from Department of Biotechnology, Government of India (File No. BT/EB/PAN IIT/2012) to undertake this work is duly acknowledged.
Author information
Authors and Affiliations
Corresponding author
Editor information
Editors and Affiliations
Rights and permissions
Copyright information
© 2019 Springer Nature Switzerland AG
About this chapter
Cite this chapter
Jadhav, D.A., Neethu, B., Ghangrekar, M.M. (2019). Microbial Carbon Capture Cell: Advanced Bio-electrochemical System for Wastewater Treatment, Electricity Generation and Algal Biomass Production. In: Gupta, S., Bux, F. (eds) Application of Microalgae in Wastewater Treatment. Springer, Cham. https://doi.org/10.1007/978-3-030-13909-4_14
Download citation
DOI: https://doi.org/10.1007/978-3-030-13909-4_14
Published:
Publisher Name: Springer, Cham
Print ISBN: 978-3-030-13908-7
Online ISBN: 978-3-030-13909-4
eBook Packages: Earth and Environmental ScienceEarth and Environmental Science (R0)