Abstract
Multibeam echosounders have revolutionized our ability to resolve seabed geomorphology and regionally define its substrate. Using a fan of narrow acoustic beams, the slant ranges, angles and returned backscattered intensity can be employed to define the seabed elevation and infer the substrate. A corridor of data with a width proportional to the projected angular sector is acquired along a vehicle track. Before interpreting the resulting geomorphology, however, it is critical to understand the practical resolution and accuracy limitations that result from a particular system configuration. The spatial resolution of the data depend on a combination of the pulse bandwidth, the projected beam widths and their resulting spacing and stabilization. For a given configuration, the single biggest factor controlling the resulting resolution is the altitude of the platform. For surface mounted systems this results in degrading definition of morphology with depth. As multibeam systems improve, there is the potential to monitor temporal changes in submarine geomorphology. To do so, however, each survey has be positioned to a standard finer than the expected change. The total propagated uncertainty in the location of the resolved topography is a combination of the uncertainty in the position and orientation sensors and their integration as well as the bottom detection algorithm and sound speed field. When interpreting apparent change, it is critical to comprehend the realistic achievable accuracies.
Access provided by CONRICYT-eBooks. Download chapter PDF
Similar content being viewed by others
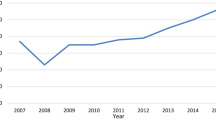
1 Introduction
1.1 Review and History
Multibeam sonar systems have become ubiquitous with underway marine geological operations. They have now been available to the scientific community for almost four decades. The first operational system was developed for the US Navy in 1964 (Glenn 1970) and by 1977 the first declassified version was available for scientific submarine geomorphological studies (Farr 1980). Since that time, the academic fleets have progressively adopted hull mounted multibeam systems as a standard tool and their use has revolutionized our understanding of seafloor processes.
All these early systems were developed for deeper water using frequencies ranging from 12–30 kHz. The first really shallow water multibeam (500–1000 kHz), was developed in 1984 for offshore oil and gas infrastructure support (Hammerstad et al. 1985). Since that time these shorter range systems have increasingly been adopted to define coastal and shelf submarine geomorphology (Hughes Clarke et al. 1996).
The original driving force behind the development of multibeam sonar arose from military requirements to navigate submarines and ballistic missiles over ocean basins using inertial sensors. Bathymetric terrain matching allowed submarines to update their position without surfacing. Deflections of the gravity vector due to large scale seabed relief such as seamounts were a significant source of error for inertial navigation. The technology was rapidly adopted to support the laying of both military and commercial deep sea cables. As an incidental byproduct of the surveying, it became apparent that there was much to be learnt about seabed morphology at lengths scales above a few hundred metres. This length scale reflected the resolvable topography at those depths and matched the best capable offshore navigational accuracy of the time (transit satellites and single point GPS).
The incentive to develop these systems in continental shelf depths was driven by the increasing exploitation of offshore oil and gas fields. At shorter ranges, features could now be resolved down to wavelengths less than 10 m. As such it placed increasing demands on navigational accuracy. Near coastal navigation systems such as Decca and Loran were not adequate, but as Differential GPS was developed this became feasible. Over the past two decades, the parallel improvements in positioning and sonar technology have continued to drive down the positioning uncertainty and minimum resolvable dimensions. This has opened up new applications in submarine geomorphology.
1.2 Current Uses in Submarine Geomorphology
As the early systems were all developed for oceanic depths, the immediate benefit was primarily for deep ocean morphology ranging from mid ocean ridge fabric to continental margin mass wasting. The great advantage over single beam sounding was that the observer could now examine a three dimensional surface rather than interpolate between sparse two dimensional profiles. This allows the discrimination of lineaments in azimuth, something that previously had not been possible without subjective extrapolation. With a 3D surface, the directional roughness spectrum could now be directly measured (Fox and Hayes 1985). At the same time it became apparent that geomorphic interpretation had to be tempered by knowledge of the limitations inherent in the multibeam systems themselves (de Moustier and Kleinrock 1986).
For the first 20 years of use, given the paucity of preceding data, the main aim was to define the current state of the seafloor. Beginning in the early 90’s as survey density increased, interest arose in the ability to resolve seabed change.
The first example of this for change detection was Fox et al. (1992). They were able to recognizing the localized expression of 50 m depth changes (in 2000 m of water) due to fresh lava flows. The lateral extent of the flows were several hundred metres, allowing identification even with the poor positioning confidence of the time.
2 Physical/Technical Principles of the Method
2.1 Imaging Geometry
A multibeam sonar consists of a pair of orthogonally mounted linear acoustic arrays. The transmitter (Tx) is usually oriented along the fore-aft axis of the bottom of the vessel whereas the receiver (Rx) is mounted athwartship. The system may be broken into its transmit geometry and reception geometry (Fig. 1). The initial description herein reflects a single sector multibeam.
On transmission, a corridor across track is illuminated with a beam that is narrow in the fore-aft direction. Using the receiver, multiple channels are simultaneously formed from beams which are spaced at different across-track steering elevations (Fig. 1c). When the illumination pattern on the seafloor is matched with the reception pattern, a series of small beam footprints are formed. Within each of those footprints, a slant range to the seabed can be estimated and the backscattered intensity at that point can be measured. Using the imaging geometry (azimuth and depression angle of the beam) and correcting for the refracted ray path, a depth at each footprint can be estimated. And if the received intensity is corrected for geometry (ensonified area and range) and radiometry (source level and beam patterns), an estimate of the bottom backscatter strength may be derived (de Moustier 1986) which is strongly correlated with the seabed composition (see also Chapter “Sidescan Sonar”).
A bathymetric and bottom backscatter map can thus be collated by combining these data collected along a swath on either side of the ship’s track (Fig. 2). As the swath is defined by an angular sector, the swath width usually grows linearly with depth. Similarly, as the solution density reflects the projection of beams spaced at different angles across the swath, the achievable resolution of the system will also degrade linearly with depth.
2.2 Range Performance
As multibeams are most commonly used from the surface, the required range performance will vary from full ocean to coastal depths. Depending on the depth in the area of interest, the sonar frequency choice needs to be optimized. Range performance of a system is based on maintaining sufficient signal to noise ratio (SNR). The range dependent component of this has both a geometric component (spherical spreading) which is frequency independent and a path length attenuation component which is strongly frequency dependent (Francois and Garrison 1982). As a result lower frequencies, which have lower attenuation coefficients, are utilized in deeper water.
The total achievable range is dependent on the source level, the frequency dependent attenuation coefficient, the scattering properties of the seafloor, the length of the pulse utilized and the background noise levels. Most sonars operate at their maximum source level and thus the only operator-controlled variable is the pulse length. As the pulse length controls the ensonified area (colour bands in Fig. 1 lower left), as a sonar system goes deeper, the pulse length is usually increased to maximize the signal to noise.
The net result of all these factors is that a performance envelope plot (Fig. 3) can be generated that predicts the useable depth and swath width coverage that can be achieved for a given sonar frequency. Note that the swath width initially grows linearly with depth until the outer most beam bottom detections drop below the usable SNR. Beyond that depth, the advertised maximum angular sector of the sonar can no longer be obtained and the total swath width in metres generally slowly shrinks with depth. Those bottom detections close to the SNR limit will exhibit higher range uncertainty.
Given a specific sonar configuration, the environmental factors that affect range performance are noise conditions and seafloor geology. Plots like Fig. 3 usually provide a family of curves depending on seabed type (gravel, sand, mud) and assume a specific noise level. While there are environmental factors that control noise in the ocean, one of the largest factors is vessel self-generated noise. One particular factor is flow noise and engine/propeller noise. Both of these usually increase with speed. The net result is, to achieve the intended swath width, the vessel can rarely steam at its full transit speed. Slowing down provides notably improved data quality. The geomorphologist should thus temper their desire to increase coverage by using higher speeds.
An ideal choice of multibeam is one in which the SNR threshold has not been reached at the outer edge of the desired swath. At the lowest frequencies commonly used (~12 kHz), this is not limiting until about 2–3000 m depth. If a wide range of depths need to be covered, however, the frequency most suitable for the deeper end of the depth range will not have the best range resolution in shallower depths. We thus have to consider the tradeoff between range performance and range resolution.
2.3 Range Resolution
The ability to discriminate targets in range (time) is a product of the bandwidth of the transmitted pulse. For a simple continuous wave (CW) pulse in which the carrier frequency is not varied, the effective range resolution corresponds closely to the pulse length. In this case the bandwidth of the pulse is approximately the reciprocal of the time duration of the pulse length. The shortest pulses that most sonars can generate are about 10 wavelengths long (equivalent to having a bandwidth of 1/10 of the centre frequency). Thus low frequency sonars usually have poorer range resolution. For example a deep water 12 kHz sonar typically has a best range resolution of 1–2 m. For this reason, as a survey moves into shallow water, and the range performance is no longer needed, higher frequency sonars are preferred.
Since ~2005 frequency modulated (FM) pulses have been introduced that maintain high bandwidth even with longer pulses. This has meant that the higher frequency systems can now be used in deeper water (see 100 kHz FM example in Fig. 3). As a result even higher frequency coastal sonars (200–400 kHz) are now being used on the shelf and 30 kHz systems are being used to full ocean depth.
As Fig. 3 indicates, there is a natural progression from the continental shelf to the full ocean depths of switching between multibeams of progressively lower centre frequencies as the tradeoff between range resolution and range performance is optimized. The next system component to consider is the angular resolution.
2.4 Angular Resolution
The angular resolution is a combination of the achievable beam width and the potential to discriminate angles within a single beam. The width of an unsteered sonar beam is approximately inversely proportional to the number of wavelength along the length of the linear arrays. For example a 1° beam (with reasonable sidelobe suppression) requires about 60–70 wavelengths. Thus a typical deep water 1° 12 kHz multibeam has a transmitter that is about 8 m long. A 30 kHz system requires about 3.5 m and a 100 kHz system needs only 1 m.
Larger arrays are both more expensive and require more available space on the bottom of the hull. Thus many multibeam systems come in a choice of array sizes depending on the surveyors need for angular resolution. Figure 4 illustrates the effect of varying beam width on the definition of a seabed target (in this case an object that subtends ~0.75° at that depth).
Varying resolution as a function of beam width. Scour depression around targets in 760 m of water. 100 m wide, 5 m deep depressions in which a ~10 m wide target is centred. In each pass the utilized length of the transmitter and receiver arrays is altered to illustrate the effect of projected along and across track beam width on the target definition
The simple relationship described above only applies to an unsteered beam. As the beam is steered its width grows inversely with the cosine of the steering angle. Thus, for example, beams steered at 60° are twice as wide. As most multibeam receivers are mounted horizontally that means that the beam width fattens away from nadir. Combined with the fact that the slant range to the seafloor is greater at oblique angles, and the beam subtends an oblique angle on the seabed, it can be appreciated that the across-track beam footprint (Figs. 1 and 5) grows rapidly toward the edge of the swath.
2.5 Bottom Detection
Given a specific range resolution and angular resolution, the multibeam needs to estimate the slant range within each projected beam footprint. A variety of methods are employed depending on the grazing angle of the beam.
Beams that are oriented close to normal incidence generally employ an amplitude detection method based on finding the centre of the echo envelope (indicated in Fig. 5). This results in an estimate of an average depth over an area the size of the whole beam footprint (defined by the projected across and along track beam widths, Fig. 1b). At lower grazing angles, the projected beam footprint rapidly becomes very large resulting in poor amplitude detection results. In this case split-aperture methods based on differential phase are preferred (Pohner and Lunde 1990). These provide an estimate of the depth over an area that now has an effective across track dimension much narrower than the physical across track beam size (Fig. 5). This is because it is based on the ability to discern the point of zero phase crossing (Fig. 5) rather than the actual duration of the echo envelope. In this way, across track morphologic resolution can be maintained. A detailed review of bottom detection algorithms is provided in de Moustier (1993).
2.6 Sounding Density
To describe a certain minimum spatial wavelength on the seafloor, depth solutions have to be at least spaced at less than half that dimension (the Nyquist criteria). Additionally the effective projected beam footprint should not exceed that same half wavelength criteria in order that relief at that scale not be filtered out.
Even if these conditions are met, however, as each beam range solution has uncertainty, the minimum amplitude of that desired seafloor roughness will be overprinted by the pseudo random bottom detection noise. To alleviate this it helps to overlap the individual bottom detection footprints to get multiple redundant observations. Under these conditions, spatial averaging of the solutions by gridding will suppress the uncorrelated sounding noise but preserve the resolvable relief, thereby providing a better view of the short wavelength seabed morphology.
The amount of overlap between beam footprints depends on the along and across track beam spacing. The across track spacing is generally controlled by the desired swath sector. As the multibeam has a finite number of beam forming channels, the wider the requested sector, the less the noise suppression. In addition to the speed of the vessel, the along track spacing depends on the acoustic travel time to the outermost beams and back. Thus again the wider the swath angular sector the less the noise suppression.
Thus by bringing in the angular sector, both the across and along track beam overlap can be increased. This will markedly improve the definition of the seabed targets. Figure 6 illustrates a pair of small volcanic edifices which are imaged by the same sonar varying both the incidence angle and the swath sector. As can be seen, by selecting narrow sectors, the definition of the relief on the edifice improves.
Varying resolution as a function of angular sector and incidence angle. The same sonar is used with ±65°, ±45° and ±25° sectors. The same pair of knolls are viewed using each sector width and from a range of incidence angles. A 125 m cube is illustrated for scale (2.5% of depth) and the contours are at 5 m intervals. Note particularly the presence of a secondary peak on the southern knoll
3 Integrated Sensors
The sonar system can only provide range and angle discrimination within a coordinate system oriented relative to the two arrays. That is only of value when integrated with the 3D position of the sonar, its orientation and any subsequent modification of the beam vector in the heterogeneous ocean medium.
The detailed calculations to perform this integration are beyond the scope of this chapter. The curious geomorphologist should, however, be aware of the steps involved as imperfections in these steps will impact the resulting topographic surface producing both static biases as well as periodic undulations. These false deviations will degrade the morphology thereby obscuring the real relief. Additionally, if repetitive surveying is the aim, these biases and false oscillations will become embedded in the apparent seabed change map obscuring the signature of real sedimentary processes (Hughes Clarke 2012).
3.1 Vessel Reference Frame
In order to relate ancillary position and orientation measurements to a sonar, all of the position, orientation and sonar sensors needs to be located and oriented within a common vessel reference frame (VRF, Fig. 7a), which is fixed within the vessel and centred on a reference point (RP). Additionally, the relative timing (latency) between sensor outputs must be established.
Errors in the offset vectors are most critical in shallow water as they represent a significant percentage of the total range to the seabed. Angular errors are equally important irrespective of depth as the distortion of the resulting beam vector (Fig. 7b) produces a depth error that is a fixed percentage of the slant range.
Standardized field calibration procedures [the Patch Test (Godin 1998)] are used to estimate the sensor to sensor angular misalignments and time latency. Failure to constrain these errors will result in biases in the geomorphology and impact estimates of seabed change.
3.2 Orientation
The orientation, measured by the inertial measurement unit (IMU) is used in two steps. First, the orientation is required to account for the rotation of the lever arm between positioning source and sonar (Fig. 7a). This is a combination of (1): the instantaneous orientation of the IMU at transmit time and receive time and (2): the mount angle of the IMU with respect to the VRF. Once the sonar location is known, the next step requiring orientation is to calculate the beam vector from the sonar in both azimuth and depression angle relative to the local level (Fig. 7b). This is a combination of all of (1) the instantaneous orientation of the VRF at transmit time and receive time (2) the mounting angles of the Tx and Rx in the VRF and (3) the sonar-relative beam vector.
Orientation angle accuracy is now feasible within 0.02° (1σ). Of greater concern is usually the accuracy with which the IMU, Tx and Rx mount angles in the VRF are known. These can result in systematic biases in the resulting swath.
Given the rapid rate of change of orientation, any time latency in the motion output relative to the sonar clock will result in periodic distortions of the resulting swath (“wobbles”, Hughes Clarke 2003). Both the static biases and the periodic errors will distort the morphology and overprint the apparent seabed change.
3.3 Horizontal Positioning
With the arrival of the GPS constellation, and now other satellite constellations (e.g.: GLONASS), surface platform positioning confidence at the antenna location can range from a few metres (Differential GPS) to a few decimeters (Precise Point Positioning) to a few centimetres (Kinematic GPS).
Legacy multibeam data acquired prior to 1990 may have used transit satellite positioning with a horizontal uncertainty in the 200+ m range. Data acquired using single point GPS prior to 2004 would have been degraded by Selective Availability which limits the positioning to ~50 m. Given the size of a beam footprint in the deep ocean, such errors can be tolerated when examining morphology at those depths.
For continental shelf surveys, however, which only really started in the 1990s, little useful data would have been acquired that was not at least Differential GPS and thus positioning within 2 m could be reliably achieved.
For more recent precise inshore multibeam surveys, in which the beam footprint is less than a metre, correspondingly higher antenna positioning accuracy would be required. To take full advantage of that level of accuracy, the VRF and its instantaneous orientation are essential to determine the unique trajectories of the sonar sensors (Fig. 8a).
3.4 Vertical Positioning
The sonar-relative depth provided by the multibeam has the vertical trajectory of the sonar embedded into it (Fig. 8b). In order to assign a meaningful depth, a vertical datum needs to be defined against which that sonar-relative elevation is referenced. Common options include mean sea level (MSL), the Geoid, Chart Datum, and the Ellipsoid. The first three are water level based and thus will be described together. The fourth datum is hard to practically realize but, as will be explained has significant advantages in reproducibility.
To reduce to the chosen datum requires a measure of the instantaneous relative offset of the sonar. That offset can be broken down into different motion periods. At the highest frequencies, the sonar is moving up and down with motions around the ocean wave spectrum. This can be captured using accelerometer-based heave sensing. Care must be taken to account for additional high frequency motion due to lever arm effects between the point at which the heave is reported (usually the RP) and the sonar (the induced heave).
For all the water level datums, once the short period motion is removed, the offset between the RP and the wave free water surface (the RP draught) needs to be estimated (CRS: Fig. 8b). This has both static and dynamic effects resulting from vessel loading and speed through the water. The remaining step is to measure the height of the wave free surface relative to the datum. This long period signal is dominated by tidal frequencies. As the amplitude and phase of the tide varies spatially this can be the single largest source of error for water level based vertical referencing.
An alternate to water level based referencing is to use the coordinate system that GPS is inherently measured in. The horizontal positioning is already WGS84 referenced, but an antenna height relative to that ellipsoid is also available (ERS: Fig. 8b). The offset between the geoid or mean sea-level from the ellipsoid varies, however by over a 100 m globally. Thus that offset needs to be measured separately (if required). If, however, the aim of the survey is seabed change, then if both surveys are ellipsoid referenced, they can be compared directly and all the imperfections in heave, draught and tide measurement are avoided completely.
3.5 Sound Speed
The final step in the integration is to account for the refracted ray path of the beam vector through the heterogeneous sound speed structure in the ocean (Fig. 7c). The sound speed is controlled by the temperature and salinity structure which varies due to oceanographic forcing. To correct for refraction, a vertical profile of the sound speed must be obtained which usually involves stopping the vessel. The challenge facing the surveyor is to decide how frequent that should be as it represents time lost toward expanding the coverage. To address this, the operator should be cognisant of the likely spatial and temporal variability in the local oceanography.
The application of sound speed involves two steps. Firstly, the array-relative beam steering requires knowledge of the speed of sound at the transducer face. Ideally there is a probe permanently mounted beside the transducers to update this continuously. This dictates the sonar relative beam vectors that are used in Sect. 3.2 to arrive at the local-level relative beam vector (Fig. 7b). The second step involves accounting for the refracted path as that ray vector leaves (and returns to) the transducer through a medium in which sound speed is known to be variable (Fig. 7c).
Imperfections in the sound speed reduction result in a depth error that grows non-linearly with across track distance. For the first step, this depth error is time varying as the vessel rolls and thus results in periodic outer swath oscillations (Hughes Clarke 2003). The second step results in a systematic bias with the across track profile either curved upward or downward (commonly referred to as “smiles and frowns”). Both of these effects degrade the resulting geomorphology.
4 State of the Art Tools
Position and orientation sensors have become increasingly reliable and accurate as well as affordable. To meet that improved positioning capability, higher resolutions are always in demand. For the sonar systems, the most common improvement has been narrower beams (culminating in the typical 0.5°–1.0° beam widths seen today), more beam forming channels, and better bottom detection algorithms.
Given all of that, the remaining limitations in sonar resolution are mainly dictated by sounding density. Two principal factors limit even and high density sounding solutions: yaw perturbations and speed limitations. Both these can be solved if the older single transmit sector is broken down into multiple sub-sectors across track which can be independently steered (Fig. 9 left). This allows active yaw stabilization which ensures that yaw perturbations of the vessel no longer result in gaps in seafloor coverage. As an extension of this concept if those sectors are duplicated fore-aft (dual swath), one can generate more than one across track profile in a single ping cycle allowing doubling of the along track data density with resulting improved resolution (Fig. 9).
Resolution and coverage benefits of dual swath. Three passes are compared over the same seafloor with ~25 cm high, 8 m long dunes (circled) developed on the back of larger dunes in 170 m of water. With single swath, the features are lost using a standard ±65° sector. To see them either requires pulling in the swath to less than half the width, or adding the second swath
State of the art multibeam echosounders nowadays also feature the “water column imaging” technique which adds the ability to detect features in the water column, such as bubble streams. While this is a direct indicator of seafloor processes, it is not strictly submarine geomorphology, and thus is not included in this chapter.
5 Strength and Weaknesses of the Method for Investigating Submarine Geomorphology
All the aspects explained above should aid the geomorphologist in predicting the minimum resolvable dimensions and with what confidence they can be positioned. This will impact the design of the multibeam survey which is always a compromise between desired resolution and areal extent. Thus the first thing to decide upon is the intent behind the survey. Specifically what is the smallest dimension of feature that needs to be resolved to address the geomorphic analysis, and what (if any) scale of change are you expecting?
Modern surface-mounted multibeam systems permit resolution down to a minimum horizontal wavelength of ~2% of altitude. Each step higher in resolution requires bringing the sonar closer to the seabed. If the required altitude is less than the depth, then submerged vehicles have to be utilized. A submerged vehicle does not enjoy the advantages of continuous satellite position updates. The relative impact of additional position uncertainty due to submergence will be discussed in Chapter “ROVs and AUVs”. In short, the gain in resolution has to be balanced against the potential loss in accuracy.
Assuming the only option is a surface vessel, there are tradeoffs that can be made in order to balance coverage and resolution/accuracy. Given the slow and fixed speed of a survey vessel, the rate of areal coverage is mainly dependent on the angular sector employed. A wider sector allows greater coverage but there are a number of compromises in achieved resolution and accuracy.
For a given angular sector, the projected beam grows nonlinearly with obliquity which results in both larger footprints and associated higher bottom detection noise. Similarly, integration errors generally grow with increased obliquity. And with wider sectors, the across track beam spacing is wider and the along track beam spacing is sparser both of which result in less footprint to footprint overlap. All of these conspire to decrease the noise suppression and hence the effective resolution.
If the intent of the survey is to monitor changes in the resolved morphology through successive surveys, then accuracy will be critical. As discussed (in Sect. 3.3), the total propagated uncertainty reflects the choice of positioning and orientation sensors, their integration, the bottom detection and the refracted ray imperfections. For accuracy, it is primarily the systematic biases due to poor lever arms, imperfect mount angles, tidal errors and incorrect water column that dominate the accuracy. Again many of these contributions compound toward the edges of the swath.
In summary, while it may be attractive to cover more ground with a wider angular sector, the quality of the data, both in resolution and accuracy can be compromised. Narrower angular sectors provide both better along and across track data density as well as higher accuracy in bottom detection registration.
6 Conclusions
Multibeam echosounders are one of the most effective ways of resolving and locating seabed geomorphology. A submarine geomorphologist would ideally like both sufficient resolution to identify the signature of active land forming processes, as well as sufficient areal extent to understand the lateral variability in that signature. For a given sonar configuration, however, the resolution is predominantly a function of projected range which, for a surface mounted system, decays with depth. At the same time though, for a fixed angular sector, areal coverage can grow with depth. This benefit should be weighed against the option of constraining the angular sector to help maintain higher sounding density. Proper interpretation of the resulting resolved morphology relies on understanding how these factors can impact the achieved resolution.
The total achievable accuracy, from which you can start to estimate seabed change, is primarily a product of the integration of sensors comprising horizontal and vertical positioning, orientation and sound speed. The uncertainty in all of these can be minimized through proper calibration of alignments, offsets and latencies, as well as frequent sound speed updates and realistic choice of swath width. Understanding what realistic scale of resolvable change is achievable is critical when interpreting apparent geomorphic change.
References
De Moustier C, Kleinrock MC (1986) Bathymetric artifacts in sea beam data: how to recognize them, what causes them. J Geophys Res 91(B3):3407–3424
De Moustier C (1986) Beyond bathymetry: mapping acoustic backscattering from the deep sea floor with Sea Beam. JASA 79(2):316–331
De Moustier C (1993) Signal processing for swath bathymetry and concurrent seafloor acoustic imaging. In: Moura and Louttie (eds) Acoustic signal processing for ocean exploration, pp 329–354
Farr HK (1980) Multibeam bathymetric sonar: sea beam and hydrochart. Mar Geodesy 4(2):77–93. doi:10.1080/15210608009379375
Fox CG, Hayes DE (1985) Quantitative methods for analyzing the roughness of the seafloor. Rev Geophys 23:1–48. doi:10.1029/RG023i001p00001
Fox CG, Chadwick WW Jr, Embley RW (1992) Detection of changes in ridge-crest morphology using repeated multibeam sonar surveys. J Geophys Res 92(B7):11149–11162
Francois RE, Garrison GR (1982) Sound absorption based on ocean measurements: part I: pure water and magnesium sulfate contributions. JASA 72:896–907
Glenn MF (1970) Introducing an operational multi-beam array sonar. Int Hydrogr Rev 47(1):35–39
Godin A (1998) The calibration of shallow water multibeam echo-sounding systems, M Eng. Report, Department of Geodesy and Geomatics Engineering Technical Report No. 190, University of New Brunswick, Fredericton, New Brunswick, Canada, 182 pp
Hammerstad E, Lovik A, Minde S, Krane L, Steinset M (1985) Field performance of the benigraph high-resolution multibeam seafloor mapping system. IEEE OCEANS’85, pp 682–685. doi:10.1109/OCEANS.1985.1160138
Hughes Clarke JE (2003) Dynamic motion residuals in swath sonar data: ironing out the creases. Int Hydrogr Rev 4(1):6–23
Hughes Clarke JE (2012) Optimal use of multibeam technology in the study of shelf morphodynamics. IAS Special Publication # 44. Sediments, Morphology and Sedimentary Processes on Continental Shelves, pp 1–28
Hughes Clarke JE, Mayer LA, Wells DE (1996) Shallow-water imaging multibeam sonars: a new tool for investigating seafloor processes in the coastal zone and on the continental shelf. Mar Geophys Res 18:607–629
Pohner F, Lunde EB (1990) Hydrographic applications of interferometric signal processing: proc. FIG XIX congress, commission 4, Helsinki
Author information
Authors and Affiliations
Corresponding author
Editor information
Editors and Affiliations
Rights and permissions
Copyright information
© 2018 Springer International Publishing AG
About this chapter
Cite this chapter
Hughes Clarke, J.E. (2018). Multibeam Echosounders. In: Micallef, A., Krastel, S., Savini, A. (eds) Submarine Geomorphology. Springer Geology. Springer, Cham. https://doi.org/10.1007/978-3-319-57852-1_3
Download citation
DOI: https://doi.org/10.1007/978-3-319-57852-1_3
Published:
Publisher Name: Springer, Cham
Print ISBN: 978-3-319-57851-4
Online ISBN: 978-3-319-57852-1
eBook Packages: Earth and Environmental ScienceEarth and Environmental Science (R0)