Abstract
Light rare earth (REE) titanates, such as REE2TiO5, REE2Ti2O7, and REE4Ti9O24, are potential matrices for the REE-actinide fraction of high-level waste from the reprocessing of spent nuclear fuel. The data on the “solubility” of impurity elements (zirconium, uranium, and calcium) in these phases are summarized. The structures considered demonstrate limited isomorphism with respect to these elements, according to the reaction 2REE3+ = Ca2+ + U4+, which is common for natural minerals and their synthetic analogues. The reasons for the low “solubility” of these impurities in the REE titanates are considered. The role of the crystal-chemical factor in the selection of matrices for the immobilization of the REE-actinide fraction is analyzed.
Similar content being viewed by others
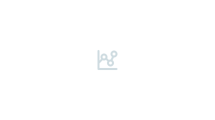
Avoid common mistakes on your manuscript.
Russia is implementing two-component nuclear power based on both thermal-neutron and fast-neutron reactors with reprocessing of spent nuclear fuel [1]. Radioactive waste, including high-level waste (HLW), originates from the nuclear fuel cycle. The development of the methods of handling long-lived transuranium actinides (Pu and minor actinides, Np, Am, Cm) is one of the key tasks in improvement of nuclear power safety. Minor actinides can be extracted from the HLW in the form of a REE-MA fraction (MA = Am, Cm) and incorporated in the crystalline phases with low solubility in water for subsequent geological disposal [2]. The REE-MA fraction mostly consist of large lanthanides (La, Ce, Pr, Nd, Sm); Am and Cm account for about 10 wt % [3]. Crystalline REE titanates and zirconates are the most promising matrices for the REE-MA fraction of SPF HLW [1, 2, 4]. Most studies have been devoted to oxides with the structures of pyrochlore and fluorite [2, 4, 5]. Fewer works are available on the properties of the REE titanates. They mainly concern the influence of the REE cations of the structure type of the REE2TiO5 and REE2Ti2O7 phases and the study of their resistance to ion irradiation [6, 7].
Nd compounds are of great interest in the search for REE-MA matrices because Nd3+ is a chemical analogue (imitator) of Am3+ and Cm3+. The following phases occur in the Nd2O3–TiO2–ZrO2 system [8]: pyrochlore Nd2(Ti,Zr)2O7 (hereafter, LnTZ), TiO2 (T, rutile), ZrTiO4 (ZT, shrilankite) and tetragonal ZrO2 (Zt). Nd-titanates are represented by Nd2TiO5 (LnT), Nd2Ti2O7 (LnT2), Nd2Ti4O11 (LnT4), and Nd4Ti9O24 (Ln2T9). Among other REE titanate and zirconate systems, La2O3–TiO2–ZrO2 [9], Y2O3–TiO2–ZrO2 [10], and Nd2O3–TiO2 [11] were studied. The identity of Nd2Ti4O11 and Nd4Ti9O24 were proven, and the occurrence of Nd2Ti3O9 (LnT3) was established in [11].
The La2О3–TiО2–ZrО2 system contains the following phases (Fig. 1b): La2TiO5 (LnT), La4Ti3O12 (Ln2T3), La2Ti2O7 (LnT2), La4Ti9О24 (Ln2T9), La2Zr2О7 (LnZ2), ZrTiО4 (ZT), ZrO2 (Z), and TiO2 (T). The phase sets in the systems with Nd2O3 and La2O3 are similar. However, the phase relations in the systems considered are different, mostly due to the much smaller area of the La–Zr-pyrochlore (LnZ2) solid-solution field. Weak variations in the chemical composition are a specific feature of Nd and La titanates. The Ti/REE ratio in the phases is close to stoichiometric values, which is manifested in the narrow stability fields in the diagrams. Less than 2 mol % of ZrO2 dissolves in the LnT2, Ln2T3, and LnT phases at 1350оС [9], while Ln2T9 contains up to 4 mol % of ZrO2 (Table 1). La2Zr2О7 (LnZ2) contains up to 35 mol % of La2O3 and 69 mol % of ZrO2, while the ideal stoichiometry suggests 33 and 67 mol % of La2O3 and ZrO2, respectively. Isomorphic substitutions of La3+ into ZrO2 and ZrTiО4 are limited by 1 mol % of La2O3. High contents (12–14 mol %) of ZrO2 in (Ti,Zr)O2 and TiO2 in (Zr,Ti)O2 were observed. The Zr/Ti ratio in ZrTiO4 varies in a wide range (1.4–0.9). Substitution of large REE3+ (La, Nd) for Y3+ leads to the transformation of the phase diagram (Fig. 1c). The Y2Ti2O7 displays a cubic symmetry (pyrochlore like) and a large solid solution field of (Zr,Y)O2 – x fluorite appears in the phase diagram. Oxides TiO2, ZrО2, and ZrTiО4 are preserved. The Y2TiO5 turns to a cubic modification (sp. group \(Fm\bar {3}m\)), while Nd2TiO5 and La2TiO5 belong to the orthorhombic symmetry (sp. group Pnma).
Variations of the REE3+ radii in the REE2О3–TiO2 and REE2О3–ZrO2 lead to polymorphic transitions. The REE2Ti2O7 and REE2Zr2O7 compounds form three structure types—fluorite, pyrochlore, and perovskite-like monoclinic titanate [3, 5–7]. The pyrochlore stability field falls to the Shannon radii ratio REE/Ti(Zr) from 1.46 to 1.78. Below 1.46 the fluorite structure type is stable, and above 1.79 the perovskite-like structure type is stable. Titanates of heavy REEs (Ln2Ti2O7, Ln = Sm–Yb, Y) and zirconates of light REEs (Ln2Zr2O7, Ln = La–Gd) belong to the pyrochlore type of structure. The oxide REE2TiO5 displays an orthorhombic symmetry (REE = La–Sm), cubic symmetry (REE = Er–Lu, Sc), and hexagonal symmetry (REE = Ho, Y) [7]. The stability of the structure type with respect to the chemical composition is an important property of the matrices. The appearance of a phase with high solubility in water during the synthesis reduces the performance of the matrices. Previously we studied the distribution of the Ca, Zr, and U impurities in the REE titanates [12–16]. The samples were synthesized by cold pressing and sintering (CPS), cold crucible inductive melting (CCIM), and melting in Joule-heated furnace and crystallization (MC) methods. Tables 2–4 and Fig. 2 list the results of our experiments. A new series of U-bearing samples (NTС-1, NTС-2, NTU-4, NTZ-1, NTZ-2, NTZ-3) was studied.
BSE images of (a) LT2 (1, LnT2; 2, Ln2T9); (b) 3b (1, LnT2; 2, UT2, brannerite; 3, (Ln,U)O2 – x; 4, REE Ti-silicate); (c) 4 (1, Ln2T9; 2, UT2, brannerite; 3, (Ln,U)O2 – x; 4, REE Ti-silicate); (d); MPM-2 (1, T, rutile; 2, Ln2T9), (e) NTC-2 (1, pyrochlore, 2, (Ln,U)O2 – x); (f) NTU-4 (1, LnT2; 2, Ln2T9; 3, (Ln,U)O2 – x). Black dots, pores. Scale bar (а, d–f) 50, (b) 20, and (c) 10 microns. The chemical compositions of the phases are listed in Tables 3 and 4.
The observed significant variations of the phase chemical compositions with respect to REE3+ (Table 3) are due to the fact that lanthanide titanates (Ln3+ = La, Ce, Nd) of the same stoichiometry (LnT, LnT2, Ln2T9) are isostructural. However, contrast to the other REE phases (pyrochlore, zirconolite, monazite, brannerite, etc.), titanates have low solubility for tetravalent (U, Zr) and divalent (Ca) elements.
The ZrO2 content is 2.0 wt % in Ln2T9 (sample MPM-2) and 8.5 wt % in rutile. The U content is 1.3 wt % in Ln2T9 (Table 3) and is below the detection limit (0.3–0.5 wt %) in LnT and LnT2 (Tables 3 and 4). The highest contents of U and Zr (>10 wt %) were found in pyrochlore (Table 4). Those peculiarities of the chemical compositions are due to the crystal chemical properties of the phases considered.
REE titanates and zirconates adopt several structure types. In most of the cases, Ti and Zr ions are six coordinated with O ions forming an octahedron. The dominant coordination numbers of large REEs (La–Sm) are VII (monocapped trigonal prism, truncated cube), VIII (cube, distorted cube, bicapped antiprism), and IX (tricapped trigonal prism). With a decrease in the REE3+ radii, the occurrence of coordination number VI increases. Description of the crystal structures of Nd3+ oxides is given in Table 5 and Fig. 3.
The Nd2Zr2O7 belongs to cubic symmetry (Fig. 3a), space group \(Fd\bar {3}m\). The unit cell contains eight formula units. The Nd coordination polyhedron is scalenohedron (distorted cube) with six equidistant O ions and two O ions at a larger distance. The Zr ion centers the trigonal antiprism (distorted octahedron). The pyrochlore structure can be considered as the framework of the BO6 corner-linked octahedra with AX2 chains filling the interstices of the octahedral framework. The pyrochlore structure is a derivative of the fluorite structure type AO2 (sp. group \(Fm\bar {3}m\)).
The Nd2Ti2O7 crystal structure is a derivative of the perovskite structure (Fig. 3b). The corner linked TiO6 octahedra form slabs four octahedra thick (~12 Å) along the directions a and b. The monocapped trigonal prisms NdO7 are in-between slabs. Tricapped trigonal prisms NdO9 fill the interstices of the octahedral slabs. Bicapped prisms NdO8 occur both inside and in-between slabs.
The Nd2TiO5 crystal structure (Fig. 3c) comprises a framework of edge-sharing monocapped trigonal prisms LnO7 and chains of vertex sharing TiO5 square pyramids along the [010] direction.
The Nd4Ti9O24 oxide has the most complex structure. It consists of a TiO6 octahedral framework, the cavities of which are filled with Nd ions (Fig. 3d). Nd ions occupy three independent positions in the structure: Nd(1) centers the distorted square antiprism, Nd(2) centers the octahedron, Nd(3) centers a distorted square prism. The Nd(1)O8 polyhedra linked by common vertices and edges form layers parallel to the (110) plane. The Nd(3) polyhedra linked by common edges with Nd(1) layers form complex secondary layers (17.5 Å thick) the cavities of which are filled with isolated Nd(2) octahedra.
The coordination polyhedra of Zr4+ and Ti4+ are octahedra (coordination number equals VI) in all the phases considered, with the exception of Nd2TiO5, where the Ti4+ coordination number equals V and the shape of the coordination polyhedron is a square pyramid. This explains the different isomorphism of elements in the phases considered, i.e., a wide field of the pyrochlore solid solution and narrow fields of phases with ratios of elements close to their nominal stoichiometry in other cases. The low solubility of Ca, Zr, and U is typical for the phases with odd Nd coordination numbers (VII and IX). It is worth noting that part of the Nd ions is eight-coordinated in Nd4Ti9O24. Probably that is the reason for the increased content of U and Zr in Nd4Ti9O24 relative to Nd2Ti2O7 and Nd2TiO5.
Narrow stability fields of La3+ and Nd3+ titanates (Figs. 1a, 1b) contrast with the wide composition variations of pyrochlore, zirconolite, and brannerite, which are also considered as matrices for the REE-actinide fraction. Zirconate and titanium–zirconate pyrochlores have a high isomorphic capacity for actinides in oxidation states other than 3+ [3–5, 20]. The Zr position of pyrochlore Nd2Zr2O7 can host up to 20 at. % U, and the Nd position can host from 10 to 20 at. % U and Th. The wide ranges of compositions of zirconolite and brannerite are due to the peculiarities of their crystal structures [3, 4]. Zirconolite CaZrTi2O7 (Fig. 4a) adopts a monoclinic symmetry (C2/m), and the Ca and Zr coordination numbers are VIII and VII, respectively. The REE and actinide cations can replace Ca, Zr, and Ti by three mechanisms. The first of them is heterovalent: Ca2+ + Zr4+ → 2M\(_{1}^{{3 + }}\) (M1 = lanthanides (Ln) and actinides (An)). At a high content of M1 cations, this structure transforms into (M1)2Ti2O7 pyrochlore. The second variant of heterovalent isomorphism Ca2+ + Ti4+ → M\(_{1}^{{3 + }}\) + M\(_{2}^{{3 + }}\) (M1 = Ln and An = Pu, Am, Cm; M2 = Al, Fe). In addition, isovalent substitution of Zr4+ for M\(_{3}^{{4 + }}\) cations (M3 = Ce, An) is possible. In zirconolite, actinides (U, Np, Pu) occur in the Ca and Zr positions in an amount of up to ~0.3 atoms per formula unit. When Zr is completely replaced by actinides, pyrochlore CaAn4+Ti2O7 is formed. Brannerite crystallizes in a monoclinic symmetry (sp. group C2/m). The U and Ti cations in the structure of brannerite are located in the centers of octahedra. The TiO6 octahedra linked by vertices and edges form layers that are parallel to the (001) plane. The columns of UO6 octahedra going along the b axis (Fig. 4b) link the layers together. Large positions are occupied by (Ce, Th, U, Np, Pu)4+ or cations of a higher oxidation state (Np5+, U5+/U6+) with simultaneous incorporation of Ca2+ and REE3+ into the structure to compensate for the charge balance, for example, by the heterovalent scheme: Ca2+ + U6+ → 2U4+ or REE3+ + U5+ → 2U4+.
Crystal structures of (a) zirconolite CaZrTi2O7 (Ti layers are gray, Ca and Zr atoms are blue and green, respectively) and (b) brannerite UTi2O6 (U octahedra (yellow) forms columns parallel to the b axis, Ti octahedra (gray) form layers along the (001) plane. U octahedra are linked with Ti octahedra by vertex sharing.
CONCLUSIONS
Using the phases of Nd (an imitator of Am and Cm), variations in the composition and crystal chemistry of possible REE-MA matrices in the Nd2O3–TiO2–ZrO2 system are considered. The system contains, in the order of decrease in the stability fields in the diagram (Fig. 1a), the following phases: Nd2(Zr,Ti)2O7, Nd4Ti9O24, ZrTiO4, (Zr,Ti)O2, (Ti,Zr)O2, Nd2Ti2O7, and Nd2TiO5. Potential matrices for the REE-MA fraction are REE2(Zr,Ti)2О7–х with the pyrochlore structure, Nd4Ti9O24, Nd2Ti2O7 (perovskite), and Nd2TiO5. Pyrochlore Nd2(Ti,Zr)2O7 has the largest stability field, which includes 10 wt % or more of U and Zr. The “solubility” of Ca, Zr, and U in Nd-titanates is low: from 2–3 wt % in Nd4Ti9O24 to below the detection limit (0.3–0.5 wt %) in Nd2TiO5 and Nd2Ti2O7. At an increased content of admixtures, U and REE oxide, U titanate (brannerite), and Ca and Zr titanate (zirconolite) appear in the matrices. The last two compounds are highly stable in solutions, and their appearance will not lead to a deterioration in the insulating properties of matrices with respect to REE-MA. In the REE2O3–TiO2 and REE2O3–ZrO2 systems, as the REE3+ radius decreases, the structure of REE2Ti2O7 compounds changes from monoclinic perovskite-like to cubic pyrochlore: the polymorphic transition boundary is between Nd and Sm. The REE2Zr2O7 phases adopt two related structure types—pyrochlore and fluorite. The first appears at a large difference between the REE3+ and Zr4+ radii (RREE/RZr > 1.46). As the difference in the ion radii decreases, the pyrochlore structure transforms into an anion-deficient fluorite (REE, Zr)4O7 structure. The phase transition boundary goes along Gd3+: zirconates of lighter and larger REEs of the Ce group belong to the pyrochlore structure type, while heavy and smaller REEs of the Y group adopt a fluorite-type structure. The situation is more complicated in the case of REE2TiO5 compounds: the REE phases from La to Sm adopt an orthorhombic symmetry, from Er to Lu and Sc they have a cubic symmetry, and the REE phases from Eu to Ho and Y are hexagonal.
Knowledge of the crystal chemical features of REE phases makes it possible to control the phase composition of the matrices. Admixtures (Zr, Fe, residual amounts of Pu, U) can either be present in the REE-MA fraction or can be specially introduced (CaO, Fe2O3, Al2O3) into the charge before the matrix is synthesized. As a result, along with REE-MA titanates, it is possible to obtain phases with the structures of pyrochlore, brannerite, and zirconolite, which do not deteriorate the properties of the matrix due to their high corrosion resistance in water.
REFERENCES
S. V. Yudintsev, Radiochemistry 63 (5), 527–556 (2021).
S. V. Stefanovsky and S. V. Yudintsev, Usp. Khim. 85 (9), 962–994 (2016).
Spent Nuclear Fuel Reprocessing Flowsheet (OECD NEA, Paris, 2012).
G. R. Lumpkin, in Experimental and Theoretical Approaches to Actinide Chemistry, Ed. by J. K. Gibson and W. A. de Jong (John Wiley & Sons, 2018), Chapter 7, pp. 333–377.
R. C. Ewing, W. J. Weber, and J. Lian, J. Appl. Phys. 95 (11), 5949–5971 (2004).
K. L. Smith, M. G. Blackford, G. R. Lumpkin, and N. J. Zaluzec, Microsc. Microanal. 12 (2), 1094–1095 (2006).
R. D. Aughterson, G. R. Lumpkin, M. Ionescu, M. de los Reyes, B. Gault, K. R. Whittle, K. L. Smith, and J. M. Cairney, J. Nucl. Mater. 467, 683–691 (2015).
S. S. Shoup, C. E. Bamberger, J. L. Tyree, and L. Anovitz, J. Solid-State Chem. 127, 231–239 (1996).
S. D. Skapin, D. Kolar, and D. Suvorov, Solid State Scie. 1, 245–255 (1999).
T. A. Schaedler, O. Fabrichnaya, and C. G. Levi, J. Eur. Ceram. Soc. 28, 2509–2520 (2008).
W. Gong and R. Zhang, J. Alloys Compd. 548, 216–221 (2013).
S. V. Yudintsev, Dokl. Earth Sci. 460 (4), 130–137 (2015).
S. V. Yudintsev, S. V. Stefanovsky, M. Yu. Kalenova, B. S. Nikonov, M. S. Nikol’skii, A. M. Koshcheev, and A. S. Shchepin, Radiochemistry 57 (3), 321–334 (2015).
S. V. Yudintsev, S. V. Stefanovsky, O. I. Stefanovskaya, B. S. Nikonov, and M. S. Nikol’skii, Radiochemistry 57 (6), 640–652 (2015).
S. V. Yudintsev, T. S. Livshits, J. Zhng, and R. C. Ewing, Dokl. Earth Sci. 461 (1), 247–254 (2015).
S. V. Yudintsev, M. S. Nikol’skii, B. S. Nikonov, and V. I. Mal’kovskii, Dokl. Earth Sci. 480 (2), 631–637 (2018).
E. J. Harvey, K. R. Whittle, G. R. Lumpkin, R. I. Smith, and S. A. T. Redfern, J. Solid State Chem. 178 (3), 800–810 (2005).
H. Mueller-Buschbaum and K. Scheunemann, J. Inorg. Nucl. Chem. 35 (4), 1091–1098 (1973).
N. Hübner and R. Gruehn, Z. Anorg. Allg. Chem. 616 (10), 86–94 (1992).
J. Sun, J. Zhou, Z. Hu, T.-S. Chan, R. Liu, H. Yu, L. Zhang, and J.-Q. Wang, J. Synchrotron. Rad. 29, 37–44 (2022).
ACKNOWLEDGMENTS
The authors are grateful to Corresponding Member of the RAS S.V. Krivovichev and an anonymous reviewer for the valuable comments that improved the manuscript significantly.
Funding
This work was supported by a State Assignment for the Institute of Geology of Ore Deposits, Petrography, Mineralogy, and Geochemistry, Russian Academy of Sciences.
Author information
Authors and Affiliations
Corresponding author
Ethics declarations
The authors declare that they have no conflicts of interest.
Additional information
Translated by M.S. Nickolsky
Rights and permissions
About this article
Cite this article
Yudintsev, S.V., Nickolsky, M.S., Stefanovsky, O.I. et al. Crystal-Chemical Considerations in the Choice of Matrices for REE-Actinides. Dokl. Earth Sc. 504, 403–409 (2022). https://doi.org/10.1134/S1028334X22060150
Received:
Revised:
Accepted:
Published:
Issue Date:
DOI: https://doi.org/10.1134/S1028334X22060150