Abstract
In this paper, 9,10-anthraquinone (AQ) derivative-modified glassy carbon (GC) electrodes were studied towards the electrochemical reduction of oxygen in aqueous and non-aqueous solutions. The reaction of 1-chloro-9,10-anthraquinone with aliphatic diamines was applied for the synthesis of amino-9,10-anthraquinone derivatives. The obtained AQ derivatives were grafted onto the surface of glassy carbon electrodes by electropolymerisation using diazonium salts. The modified GC electrodes are sensitive to oxygen, determined by cyclic voltammetry. Changes in the oxygen determination efficiency depend mostly on the length of the side chain AQ derivative which is grafted to the GC electrode and is nearly double in comparison to a bare GC electrode modified by 1-((2-((2-aminoethyl)amino)ethyl)amino)-9,10-anthraquinone.
Similar content being viewed by others
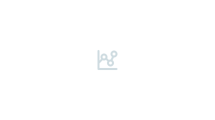
Explore related subjects
Discover the latest articles, news and stories from top researchers in related subjects.Avoid common mistakes on your manuscript.
INTRODUCTION
The development of the electronic and electrotechnical industry, the increase of environmental contamination, and in effect an increase in diversity of illness over the last few years has intensified studies on creating innovative, more sensible and efficient sensors [1, 2]. A direct reduction of analytes may be an excellent way to improve the early prevention of many diseases, including cancer [3, 4].
Additionally, monitoring the dissolved oxygen concentration plays a meaningful part in various fields, such as the food industry [5] and environmental monitoring [6]. The traditional method for dissolved oxygen determination is iodometry, which is often described as inconvenient because of its relatively long duration [7]. Another example of oxygen reduction is the Clark electrode method [8]. Unfortunately in this electrochemical technique, the oxygen is consumed during redox reaction, causing numerous restrictions in possible application [9].
Anthraquinones are commonly found in nature [10] and have found a wide range of possible applications in medicine [11, 12], as an intermediate compound for dye fabrication [13], and for hydrogen peroxide synthesis [14]. As for AQ derivatives, the isomer properties change significantly when function groups are attached [15–17]. Modifying the structure of 9,10‑anthraquinone can promote the solubility of these derivatives in aqueous and non-aqueous solutions [18, 19]. In addition, even small changes in the ring molecules can significantly affect the biological activity of the whole molecule [20–22]. 9,10-anthraquinone derivatives are used as dyes [23], and medicines such as anti-inflammatory [24] and anticancer [25] drugs.
The kinetics of oxygen reduction has been reported on a glassy carbon electrode modified with 9,10‑anthraquinone-2-ethanoic acid by anodic oxidation. Maia et al. [26] showed a reduction of oxygen at GC modified by 9,10-anthraquinone-2-carboxylic acid. Also, the one-electron reduction potentials of 116 important p- and o-quinones were estimated in DMSO using B3LYP/DZP++ quantum simulation methods by Zhu et al. [27].
In this publication, we propose a method for oxygen reduction using GC electrodes modified with amino-9,10-anthraquinone derivatives. The purpose of the present study was the fabrication and investigation of glassy carbon electrodes modified by AQ derivatives in order to achieve enhanced sensitivity versus oxygen reduction in aqueous or non-aqueous solutions. Three AQ derivatives were synthesised and electropolymerised on the GC electrodes. The reaction was based on the transformation of unbound amine groups into diazonium salts of the AQ. Compounds based on 1-chloro-9,10-anthraquinone and aliphatic amines were applied. The synthesised compounds were purified by the flash column chromatography method and subsequently described with magnetic resonance (1H-NMR) and mass spectrometry method (MALDI-TOF MS). The oxygen reduction processes were studied at AQ modified GC electrodes in aqueous and non-aqueous solutions using cyclic voltammetry.
EXPERIMENTAL
Reagents
1-Chloro-9,10-anthraquinone, was purchased from Sigma-Aldrich and used without further purification. Toluene and other solvents were obtained from Stanlab. All solvents were purified and dried using standard methods. Toluene was distilled by rectification process. The purity of the final product was verified by chromatographic methods.
Thin layer chromatography (TLC) was carried out on “TLC Silica gel 60” silica gel plates from Merck, having a particle size of 1012 μm and a sorbent thickness of 200 μm. Purification of the products was performed by flash chromatography on “Kieselgel 60” silica gel from Merck with a particle size of 0.04–0.063 mm, 230–400 mesh.
Apparatus
The electrochemical measurements involved cyclic voltammetry (CV). An Autolab, model PGSTAT 128N potentiostat was employed. All voltammetry experiments were carried out in the three-electrode system. A glassy carbon GC electrode (3 mm in diameter) was used as the working electrode, a KCl-saturated Ag/AgCl electrode served as the reference electrode, and a platinum wire was used as the counter electrode.
To identify the synthesised anthraquinone derivatives, the 1H-NMR spectra were recorded with a Bruker, AVANCE III 500 MHz spectrometer using deuterated chloroform CDCl3, IR spectra were recorded with a Bruker IFS66 spectrometer using KBr pellets, and a MALDI-TOF analysis was performed with a Brucker BIFLEX 3 spectrometer. We carried out analyses of the analysis 9,10-anthraquinone derivatives by HPLC in reverse phase (RP-HPLC) using the Shimadzu chromatograph with a UV-Vis detector and column (Phenomenex Luna C8). The analyses were performed in a flow of 1.2 ml/min of solvent gradient. (A = H2O + 0.1% trifluoroacetic acid, B = acetonitrile + 0.1% TFA).
The X-ray photoelectron spectroscopy (XPS) measurements were performed by means of Escalab 250Xi, ThermoFisher Scientific with a monochromatic AlKα source. High-resolution C1s and N1s spectra were found for the GC-modified electrodes. Pre-calibration was based on Au metal (84.0 eV). The pass energy value was 10 eV.
General Procedure for Synthesis of Amino-9,10-Anthraquinone Derivatives
1-Chloro-9,10-anthraquinone (4.15 mmol), amine aliphatic: (1a) (16.888 mmol)/(2a) (13.638 mmol)/(3a) (20.606 mmol), and anhydrous toluene (80 mL) were mixed in a 250 mL two-necked round-bottom flask equipped with a reflux condenser (see Fig. 1). The reaction mixture was stirred at 80°C for 4 days. The progress of the reaction was monitored by TLC (SiO2) in a solvent ratio of methylene chloride : methanol (1 : 0.1). After completion of the reaction, the solvent was evaporated under reduced pressure, the crude material was purified by column chromatography in the solvent ratio: pure CH2Cl2 : [CH2Cl2 : MeOH] (5 : 0.1); (1 : 0.1); (3 : 2); (1 : 1) using silica gel to afford anthraquinone derivatives (1), (2), (3). The resulting fraction with the appropriate compound was dried on a vacuum evaporator and tested by MALDI-TOF. The compound for further testing was stored in a desiccator under reduced pressure. The described general procedure for the synthesis of anthraquinone derivatives is based on the synthesis schemes described earlier in the literature [28].
Spectral Data of Anthraquinone Derivatives
Synthesis 1. (1) 1-((2-aminoethyl)amino)-9,10-anthraquinone
Yields: m = 326 mg; 27%.TLC (SiO2): CH2Cl2 : MeOH (1 : 0.1); Rf = 0.39. 1H-NMR (CDCl3): 2.85–2.88 (t, 2H, CH2–CH2–NH2, J1 = J1 = 7.0 Hz); 3.35–3.39 (q, 2H, CH2–CH2–NH2, J1 = 7.0 Hz; 5.5 Hz; 6.5 Hz, J2 = 6.0 Hz; 6.25 Hz, J3 = 6 Hz); 7.01–7.03 (d, 1H, H2-Ar, J1=8.0 Hz); 7.46–7.49 (t, 1H, H3-Ar, J1 = 7.5 Hz; 8.5 Hz; J2 = 8 Hz); 7.52–7.53 (d, 1H, H4-Ar, J1= 6.0 Hz); 7.62–7.65 (t, 1H, H6-Ar, J1 = 6.0 Hz; 9.0 Hz; J2 = 7.5 Hz); 7.68–7.70 (t, 1H, H7-Ar, J1 = J2 = 7.5 Hz); 8.16–8.18 (d, 1H, H5-Ar, J1= 8.5 Hz); 8.20–8.21 (d, 1H, H8-Ar, J1 = 8.0 Hz); 9.70 (s, 2H, ‒NH2). MALDI-TOF MS: m/z = 268 [M + 2H]+, (m0/z = 266.30 [M]). IR (KBr) (cm–1): 3430, 2956, 2923, 2853, 1740, 1679, 1660, 1593, 1577, 1465, 1443, 1426, 1376, 1353, 1314, 1279, 1262, 1236, 1218, 1175, 1134, 1084, 1054, 1029, 1011, 963, 930, 905, 828, 801, 751, 739, 728, 704. HPLC: tr = 9.69 min.
Synthesis 2. (2) 1-((3-aminopropyl)amino)-9,10-anthraquinone
Yields: m = 751 mg; 61%.TLC (SiO2): CH2Cl2 : MeOH (1 : 0.1); Rf = 0.21.1H-NMR (CDCl3): 1.63–1.81 (m, 2H, CH2–CH2–CH2–NH2); 2.14–2.34 (m, 2H, CH2–CH2–CH2–NH2), 3.40–3.64 (m, 2H, CH2–CH2–CH2–NH2), 7.48–7.50 (d, 1H, H2-Ar, J1 = 9.0 Hz); 7.60–7.63 (t, 1H, H3-Ar, J1 = 7.0 Hz; 7.5 Hz; J2 = 7.7 Hz); 7.65–7.66 (d, 1H, H4-Ar, J1 = 8.0 Hz); 7.71–7.74 (t, 1H, H6-Ar, J1 = 6.0 Hz; 7.0 Hz; J2 = 6.5 Hz); 7.75–7.78 (t, 1H, H7-Ar, J1 = 8.0 Hz; 4.0 Hz; J2 = 6.0 Hz); 8.20–8.21 (d, 1H, H5-Ar, J1= 8.0 Hz); 8.25–8.27 (d, 1H, H8-Ar, J1= 7.0 Hz). MALDI-TOF MS: m/z = 282 [M + 2H]+, (m0/z = 280.33 [M]). IR (KBr) (cm–1): 3424, 3267, 2924, 2853, 1665, 1629, 1593, 1573, 1508, 1467, 1404, 1358, 1311, 1268, 1231, 1173, 1154, 1070, 1004, 930, 870, 827, 805, 777, 734, 707. HPLC: tr = 6.25 min.
Synthesis 3. (3) 1-((2-((2-aminoethyl)amino) ethyl)amino)-9,10-anthraquinone
Yields: m = 325 mg; 26%.TLC (SiO2): CH2Cl2 : MeOH (5 : 0.1); Rf = 0.16. 1H-NMR (CDCl3): 1.45–1.49 (q, 2H, CH2–CH2–NH–CH2–CH2–NH2, J1 = 7.0 Hz; 7.5 Hz; 8.0 Hz, J2 = J3 = 7.5 Hz); 2.92–2.94 (t, 2H, CH2–CH2–NH–CH2–CH2–NH2, J1 = 7.0 Hz; 4.0 Hz; J2 = 5.5 Hz); 2.97–2.99 (t, 2H, CH2–CH2–NH–CH2–CH2–NH2, J1 = 5.0 Hz; 5.5 Hz; J2 = 5.0 Hz); 3.10–3.12 (t, 2H, CH2–CH2–NH–CH2–CH2–NH2, J1 = J2= 6.0 Hz); 3.26–3.30 (t, 2H, CH2–CH2–NH–CH2–CH2–NH2, J1 = 9.0 Hz; 8.0 Hz; J2= 8.5 Hz); 7.12–7.14 (d, 1H, H2-Ar, J1 = 9.0 Hz); 7.57–7.60 (t, 1H, H3-Ar, J1 = 8.5 Hz; 6.0 Hz; J2 = 7 Hz); 7.63–7.64 (d, 1H, H4-Ar, J1 = 7.0 Hz); 7.75–2.73 (t, 1H, H6-Ar, J1 = J2 = 6.5 Hz); 7.80–7.77 (t, 1H, H7-Ar, J1 = 7.5 Hz; 6.5 Hz; J2 = 7.0 Hz); 8.15–8.16 (d, 1H, H5-Ar, J1 = 8.0 Hz); 8.20–8.22(d, 1H, H8-Ar, J1 = 9.0 Hz); 9.85 (s, 1H, –NH). MALDI-TOF MS: m/z =311 [M+2H]+, (m0/z = 309.37 [M]). IR (KBr) (cm–1): 3380, 3269, 3066, 2961, 2874, 1666, 1629, 1592, 1509, 1462, 1407, 1381, 1307, 1271, 1232, 1174, 1068, 1010, 880, 829, 803, 737, 710. HPLC: tr = 5.91 min.
RESULTS AND DISCUSSION
Preparation and Modification of GC Electrodes
The electrochemical modification of the GC electrode surfaces was carried out in a mixture of diazonium salt of anthraquinone derivatives using a GC working electrode (see scheme in Fig. 2). In the first stage, the surface of the working electrode (glassy carbon) was polished with 0.05 µm of Al2O3 “Micropolish” powder by Buehler on a wet pad. After polishing, to completely remove the alumina from the electrode surface, the electrode was rinsed with a direct stream of ultrapure water of conductivity of ca. 0.056 mS/cm. The electrolyte was then deoxygenated for 15 min to ensure anaerobic conditions during the measurement. The last step was washing the electrode in methanol in an ultrasonic bath for 5–10 min and drying.
The functionalisation of the GC electrodes was performed by electropolymerisation using cyclic voltammetry. The electrochemical modification of the GC electrode surface was carried out in a solution of diazonium salt of anthraquinone derivatives using a GC working electrode (see scheme in Fig. 2) over 20 scans, at a scan rate 100 mV/s. The cyclic voltammetry was performed in the potential range –0.05 V and 0.6 V (vs. Ag/AgCl) in 0.5 mM \({\text{Fe}}({\text{CN}})_{6}^{{{{3 - } \mathord{\left/ {\vphantom {{3 - } {4 - }}} \right. \kern-0em} {4 - }}}}\) and in 0.5 M Na2SO4 solution at a scan rate 100 mV/s.
Each of the anthraquinone derivatives—(1) (19.443 mg, 0.0731 mmol), (2) (19.104 mg, 0.0681 mmol), and (3) (22.568 mg, 0.073 mmol)—was dissolved in 1 mL of concentrated hydrochloric acid (POCh, Poland). Next, 1 mL of deionised water was added while stirring the solution, cooled to 0°C in an ice bath. After 30 min, 12.5 mg (0.1811 mmol) of sodium nitrite in 1.5 mL of water was added dropwise to the reaction mixture and stirred in an ice water bath for 30 min. Each diazonium salt (2 mL) of anthraquinone derivatives was placed in an electrochemical cell with a GC working electrode and the potential was cycled between 0.5 V and −0.75 V for 20 scans at a scan rate of 100 mV s−1 (see Fig. 3). The modified electrodes were washed using distilled water and methanol and dried under a stream of nitrogen [29].
For each of the investigated compounds (1–3), a significant change in the position of peak current is observed during each cyclic voltammetry scan during modification (Fig. 3). For compound (1) a quasi-reversible oxidation reduction process of anthraquinone in acidic solution is observed (Fig. 3a). At the reduction part of CV at potential of ca. –0.4 V, the cathodic current significantly decreases in successive runs. In the anodic part (+0.14 V) an increase of the anodic peaks current is observed, which may suggest that the concentration of electrochemically active anthraquinone groups are anchored on the electrode surface.
The electropolymerisation process of the GC electrode surface with the derivative (2) is presented in (Fig. 3b). In this process, the cathodic peak current decreased when the electrode was cycled between a potential of –0.35 and –0.1 V.
Similar behaviour to the case of deposition by anthraquinone derivatives (2) is observed for the case of modification by compound (3) (Fig. 3c). Only decreases of the anodic current are observed. The value of observed current (around 60–300 µA) was significantly high than for compounds (1) (around 45 µA). For compounds (2) and (3), no clearly formed oxidation reduction process of anthraquinone was observed.
We expect that the anthraquinone derivatives are deposited on the surface of the electrodes as a result of the reaction of diazonium derivatives of aliphatic amino groups [30]. Compound (3) has two amino groups in an aliphatic chain that can participate in electrochemical polymerisation via their diazonium salts [31]. This is probably the cause of differentiation observed in the current values. Structural differences in the investigated compounds as well as the number of available electroactive groups are the reason for the observed differences in the values of the deposition currents during modification of GC electrodes.
Characterisation of GC Electrodes before and after Modification with Diazonium Salt Anthraquinone Derivatives—(1), (2), (3)
Each of the GC electrodes was characterised electrochemically, including via cyclic voltammetry measurements, before and after the functionalization of the the anthraquinone derivatives (1–3). The modification process included differences in: (I) the width of the electrochemical window measured in 0.5 M Na2SO4, and (II) the efficiency of the electrochemical process carried out in the solution containing 0.5 mM \({\text{Fe}}({\text{CN}})_{6}^{{{{3 - } \mathord{\left/ {\vphantom {{3 - } {4 - }}} \right. \kern-0em} {4 - }}}}\) in 0.5 M Na2SO4 used as a redox probe.
The changes between the bare GC electrode and the modified GC electrode by diazonium salts (1), (2), and (3) were investigated by cyclic voltammetry directly after the modification process.
It can be noticed that after the modification process, changes occurred in the electrochemical window width (Figs. 4a–4c). The black dotted cyclic voltammogram shows a potential window from 1.7 to –1.75 V for the bare GC electrode before modification. The range of the electrochemical potential window shifts for electrodes modified by diazonium salts of anthraquinone derivatives, as presented in Table 1. In all cases, the electrochemical potential window has a narrower range.
(a, b, c) Electrochemical windows of cyclic voltammetry measured before and after modifications of GC electrodes with diazonium salts of anthraquinone: (1), (2), (3). (d, e, f) Cyclic voltammograms measured before and after modifications of GC electrodes with diazonium salts (1), (2), (3). Measuring range +0.6 to –0.05 V vs. Ag/AgCl electrode, carried out in a standard solution containing 0.5 mM \({\text{Fe}}({\text{CN}})_{6}^{{{{3 - } \mathord{\left/ {\vphantom {{3 - } {4 - }}} \right. \kern-0em} {4 - }}}}\) in 0.5 M Na2SO4, scan rate 100 mV/s.
In order to verify the GC electrode modification by the (1), (2), (3) diazonium salts of anthraquinone derivatives, measurements in a solution containing 0.5 mM \({\text{Fe}}({\text{CN}})_{6}^{{{{3 - } \mathord{\left/ {\vphantom {{3 - } {4 - }}} \right. \kern-0em} {4 - }}}}\) in 0.5 M Na2SO4 were carried out (Figs. 4d–4f). The black dotted curves present the electrode measurements before modification. The peak-to-peak separation (ΔE) before modification for the bare GC electrode was 67 mV. After the modification process, changes in electrochemical behaviour are observed for all electrodes (Figs. 4d–4f). This phenomenon indicates that efficient electropolymerisation processes occurred on the electrode surfaces. The cyclic voltammograms presented in Figs. 4d–4f show the differences between the peak-to-peak separation (ΔE), which is 67 mV for the bare GC electrode, while after modification by compounds (1), (2), and (3), ΔE changed to 90, 99, and 131 mV, respectively. This phenomenon was observed previously by modification of different electrodes by anthraquinone derivatives. After the modification process by anthraquinone compounds, access to the active sites of the GC is more inhibited [32], and at the electrode surface, a porous anthraquinone film forms [33]. It can be noticed that after modification by diazonium salt of anthraquinone derivative (3), which contains the longest alkyl chain in the structure (–CH2–NH–Et–NH2), the value of peak-to-peak separation (ΔE) is highest. This suggests that the grafting efficiency of the electrode by compound (3) is highest.
High-Resolution XPS Spectra of Modified Glassy Carbon (GC) Electrodes
High-resolution XPS spectra reveal successful modification of the glassy carbon electrodes, as can be seen in Fig. 5. These measurements were carried out in the C1s and N1s region. In the case of C1s, the primary component at 284.7 eV originates from an overlapping signal of graphitic carbon in the electrode substrate [34] and aromatic rings in anthraquinone [35]. The second and third C1s components, positively shifted at +0.7 and +2.3 eV, contribute to the carbon-nitrogen bonds in the amine groups, and C=O bonds in the anthraquinone molecule [36]. The quantitative information may be impaired due to surface adventitious carbon contamination [37].
Importantly, the spectral deconvolution of the signal recorded in the N1s energy range proves the presence of amine groups, such as in the functionalisation layer [38]. Its amount appears to be similar for the (1) and (2) samples, but slightly higher on the surface of the (3) electrode. A detailed analysis of the deconvoluted data is summarised in Table 2.
Reduction of Oxygen at GC Electrodes Modified by Derivatives of Anthraquinone
The voltammograms of GC electrodes modified by (1), (2), (3) derivatives of anthraquinone have been studied towards oxygen reduction. All measurements were carried out in 0.5 M Na2SO4 aqueous and non-aqueous DMSO solutions without oxygen, and in saturated solutions reaching an oxygen solubility of 8 mg/dm3 and 70.4 mg/dm3, respectively [39, 40]. The obtained cyclic voltammograms are presented in Fig. 6, with the cyclic voltammograms recorded for bare GC.
All performed experiments, recorded on electrodes modified by anthraquinone derivatives, show enhanced efficiency and increased intensity of all reduction peaks both in aqueous and non-aqueous solutions.
The GC electrodes modified with compounds (1), (2), (3) under the influence of an applied voltage and a subsequent reaction with oxygen give an explicit response on the voltammograms of the reduction of O2 present in an aqueous solution [41]. The noticeable changes of peak splitting between peaks indicate the possibility of water-dissolved oxygen reduction with the modified GC electrodes. These results are also reported by Kocak and Jürmann et al. [42, 43], who report that anthraquinone-modified GC electrodes are able to catalyse the reduction of oxygen. They showed that the main product of oxygen reduction was hydrogen peroxide. In the DMSO solution, the shapes of the obtained cyclic voltammograms are different, but it clearly suggests that oxygen reacts most effectively with derivative (3)—anthraquinone derivatives containing a –CH2–NH–Et–NH2 chain in the structure.
Furthermore, the most significant changes are observed for the electrode modified by derivative (3) in a 0.5 M Na2SO4 solution (Fig. 6c). On the cyclic voltammograms obtained in a solution saturated by oxygen registered at the electrode modified by derivative (2), one cathodic peak is present which is similar to those obtained for the bare GC electrode (Fig. 6b). During measurements of electrodes modified by derivatives (1) and (3), two peaks are present on the cyclic voltammograms (Figs. 6a, 6c). In the case of the electrode modified by derivative (3), these peaks are higher, which suggests that modification by compound (3) is most effective in the reaction of oxygen.
The reduction of O2 at anthraquinone modified glassy carbon electrodes is explained by the mechanism given below to proceed through the reaction of oxygen with the anthraquinone radical anion [44–48].
The reaction is described by equation (1):
where Q corresponds to the anthraquinone species. The rate-determining step and the overall rate are determined by the surface concentration of AQ [49]. The superoxide anion can be further reduced as shown in (2) or disproportionate on the electrode surface (3):
Both of these processes are considered to have high efficiency and lead to the formation of peroxide, or undergo further reduction to H2O2 in accordance with (4):
Thus, carbon electrodes modified with quinone derivatives could be used to yield peroxide in these systems. The obtained AQ-modified glassy carbon electrode has shown high electrocatalytic activity for oxygen reduction as expected from the proposed mechanism. This fact indicates that the reduction of oxygen in a non-aqueous environment is related to the stabilisation of the radical-anion QA– and the dianion AQ2–. Essentially, in non-aqueous solutions, we observe a greater stabilisation of the anion radical [50] or/and probably the formation of a molecular complex reduced form of oxygen an anthraquinone molecule anchored on the surface of the electrode [51].
CONCLUSIONS
Summarising, various anthraquinone-modified GC electrodes were studied towards the electrochemical reduction of oxygen in aqueous and non-aqueous solutions. The reaction of 1-chloro-9,10-anthraquinone with aliphatic diamines led to various anthraquinone derivatives being obtained. The obtained (1–3) derivatives were grafted to the surface of glassy carbon electrodes using its diazonium salt.
This process was based on the reaction of primary amino groups of selected anthraquinone derivatives to their diazonium salts, followed by the addition of the anthraquinone derivatives to the GC electrode with the N2 release.
GC electrodes modified with derivatives (1), (2), (3) exhibit shifts in their electrochemical windows. After the modification of the GC electrodes changes in electrochemical windows can be noticed which proves an interaction with the oxygen in DMSO and Na2SO4 solutions.
Changes in the oxygen determination efficiency depend mostly on the length of the side chain of the anthraquinone derivative grafted onto the GC electrode. The obtained results indicate that the GC electrode modified with an AQ derivative with the –CH2–NH–Et–NH2 side chain shows the most efficient oxygen response in an aqueous solution. For this electrode, the increase of oxygen determination efficiency is doubled in comparison to a bare glassy carbon surface. In contrast, the oxygen dissolved in a non-aqueous solution (DMSO) results in the most significant changes for the electrode modified by derivative (3) of anthraquinone. This study shows that GC electrodes modified by anthraquinone derivatives are sensitive towards oxygen reaction in both aqueous and non-aqueous solutions.
REFERENCES
He, B.-S. and Zhang, J.-X., Rapid detection of ascorbic acid based on a dual-electrode sensor system using a powder microelectrode embedded with carboxyl multi-walled carbon nanotubes, Sensors, 2017, vol. 17, p. 1549.
Zarzeczańska, D., Ramotowska, S., Wcisło, A., Dąbkowska, I., Niedziałkowski, P., and Ossowski, T., In pursuit of the ideal chromoionophores (part I): pH-spectrophotometric characteristics of aza-12-crown-4 ethers substituted with an anthraquinone moiety, Dyes Pigm., 2016, vol. 130, p. 273.
Castillo, J.J., Svendsen, W.E., Rozlosnik, N., Escobar, P., Martínez, F., and Castillo-León, J., Detection of cancer cells using a peptide nanotube-folic acid modified graphene electrode, Analyst, 2013, vol. 138, p. 1026.
Niedziałkowski, P., Czaczyk, E., Jarosz, J., Wcisło, A., Białobrzeska, W., Wietrzyk, J., and Ossowski, T., Synthesis and electrochemical, spectral, and biological evaluation of novel 9,10-anthraquinone derivatives containing piperidine unit as potent antiproliferative agents, J. Mol. Struct., 2019, vol. 1175, p. 488.
Mills, A., Oxygen indicators and intelligent inks for packaging food, Chem. Soc. Rev., 2005, vol. 34, p. 1003.
Mülazımoğlu, A.D., Yılmaz, E., and Mülazımoğlu, İ.E., Dithiooxamide modified glassy carbon electrode for the studies of non-aqueous media: electrochemical behaviors of quercetin on the electrode surface, Sensors, 2012, vol. 12, p. 3916.
Zhang D., Kambe S., Ohba, Y., and Mizuguchi, H., Novel measuring and correction method of oxygen content in La2 – xSrxCuOy superconductors with the dissolved oxygen sensor, J. Ceram. Soc. Jpn., 2015, vol. 123, p. 68.
Wang, Y., Xu, H., Zhang, J., and Li, G., Electrochemical sensors for clinic analysis, Sensors (Basel), 2008, vol. 8, p. 2043.
Wolfbeis, O.S., Luminescent sensing and imaging of oxygen: fierce competition to the Clark electrode, BioEssays, 2015, vol. 37, p. 921.
Bringmann, G. and Irmer, A., Acetogenic anthraquinones: biosynthetic convergence and chemical evidence of enzymatic cooperation in nature, Phytochem. Rev., 2008, vol. 7, p. 499.
Yan, D., Ma, Y., Shi, R., Xu, D., and Zhang, N., Pharmacokinetics of anthraquinones in Xiexin decoction and in different combinations of its constituent herbs, Phytother. Res., 2009, vol. 23, p. 317.
Białobrzeska, W., Niedziałkowski, P., Malinowska, N., Cebula, Z., and Ossowski, T., Analysis of interactions between calf thymus DNA and 1,5-di(piperazin-1-yl)anthracene-9,10-dione using spectroscopic and electrochemical methods, J. Mol. Liq., 2019, vol. 289, p. 111080.
Salabert, J., Sebastián, R.M., and Vallribera, A., Anthraquinone dyes for superhydrophobic cotton, Chem. Commun., 2015, vol. 51, p. 14251.
Campos-Martin, J.M., Blanco-Brieva, G., and Fierro, J.L.G., Hydrogen peroxide synthesis: an outlook beyond the anthraquinone process, Angew. Chem. Int. Ed., 2006, vol. 45, p. 6962.
Ramotowska, S., Zarzeczańska, D., Dąbkowska, I., Wcisło, A., Niedziałkowski, P., Czaczyk, E., Grobelna, B., and Ossowski, T., Hydrogen bonding and protonation effects in amino acids’ anthraquinone derivatives—spectroscopic and electrochemical studies, Spectrochim. Acta Part A: Mol. Biomol. Spectrosc., 2019, vol. 222, p. 117226.
Kowalczyk, A., Nowicka, A.M., Jurczakowski, R., Niedzialkowski, P., Ossowski, T., and Stojek, Z., New anthraquinone derivatives as electrochemical redox indicators for the visualization of the DNA hybridization process, Electroanalysis, 2010, vol. 2, p. 49.
Zarzeczańska, D., Niedziałkowski, P., Wcisło, A., Chomicz, L., Rak, J., and Ossowski, T., Synthesis, redox properties, and basicity of substituted 1-aminoanthraquinones: spectroscopic, electrochemical, and computational studies in acetonitrile solutions, Struct. Chem., 2014, vol. 25, p. 625.
Wcisło, A., Cirocka, A., Zarzeczańska, D., Niedziałkowski, P., Nakonieczna, S., and Ossowski, T., Polyether precursors of molecular recognition systems based on the 9,10-anthraquinone moiety, Spectrochim. Acta Part A: Mol. Biomol. Spectrosc., 2015, vol. 137, p. 979.
Wcisło, A., Dąbkowska, I., Czupryniak, J., Ossowski, T., and Zarzeczańska, D., Unusual behavior in di-substituted piperidine and piperazine anthraquinones upon protonation—spectral, electrochemical, and quantum chemical studies, J. Mol. Liq., 2019, vol. 279, p. 154.
Wcisło, A., Niedziałkowski, P., Wnuk, E., Zarzeczańska, D., and Ossowski, T., Influence of different amino substituents in position 1 and 4 on spectroscopic and acid base properties of 9,10-anthraquinone moiety, Spectrochim. Acta Part A: Mol. Biomol. Spectrosc., 2013, vol. 108, p. 82.
Czupryniak, J., Niedziałkowski, P., Karbarz, M., Ossowski, T., and Stojek, Z., Lysine and arginine oligopeptides tagged with anthraquinone: electrochemical properties, Electroanalysis, 2012, vol. 24, p. 975.
Niedziałkowski, P., Ossowski, T., Majewski, R., Nowakowska, Z., and Schroeder, G., Thiol-functionalized anthraquinones: mass spectrometry and electrochemical studies, Monatsh. Chem., 2011, vol. 142, p. 1121.
Novotný, Č., Dias, N., Kapanen, A., Malachová, K., Vándrovcová, M., Itävaara, M., and Lima, N., Comparative use of bacterial, algal and protozoan tests to study toxicity of azo- and anthraquinone dyes, Chemosphere, 2006, vol. 63, p. 1436.
Choi, R.J., Ngoc, T.M., Bae, K., Cho, H.-J., Kim, D.-D., Chun, J., Khan, S., and Kim, Y.S., Anti-inflammatory properties of anthraquinones and their relationship with the regulation of P-glycoprotein function and expression, Eur. J. Pharm. Sci., 2013, vol. 48, p. 272.
William Lown, J., Anthracycline and anthraquinone anticancer agents: current status and recent developments, Pharmacol. Ther., 1993, vol. 60, p. 185.
Maia, G., Maschion, F.C., Tanimoto, S.T., Vaik, K., Mäeorg, U., and Tammeveski, K., Attachment of anthraquinone derivatives to glassy carbon and the electrocatalytic behavior of the modified electrodes toward oxygen reduction, J. Solid State Electrochem., 2007, vol. 11, p. 1411.
Zhu, X.-Q. and Wang, C.-H., Accurate estimation of the one-electron reduction potentials of various substituted quinones in DMSO and CH3CN, J. Org. Chem., 2010, vol. 75, p. 5037.
Polsky, R., Harper, J.C., Wheeler, D.R., Dirk, S.M., Arango, D.C., and Brozik, S.M., Electrically addressable diazonium-functionalized antibodies for multianalyte electrochemical sensor applications, Biosens. Bioelectron., 2008, vol. 23, p. 757.
Seinberg, J.-M., Kullapere, M., Mäeorg, U., Maschion, F.C., Maia, G., Schiffrin, D.J., and Tammeveski, K., Spontaneous modification of glassy carbon surface with anthraquinone from the solutions of its diazonium derivative: an oxygen reduction study, J. Electroanal. Chem., 2008, vol. 624, p. 151.
Breton, T. and Bélanger, D., Modification of carbon electrode with aryl groups having an aliphatic amine by electrochemical reduction of in situ generated diazonium cations, Langmuir, 2008, vol. 24, p. 8711.
Zolfigol, M.A., Habibi, D., Mirjalili, B.F., and Bamoniri, A., The use of Nafion-H®/NaNO2 as an efficient procedure for the chemoselective N-nitrosation of secondary amines under mild and heterogeneous conditions, Tetrahedron Lett., 2003, vol. 44, p. 3345.
Mooste, M., Kibena-Põldsepp, E., Marandi, M., Matisen, L., Sammelselg, V., and Tammeveski, K., Electrochemical properties of gold and glassy carbon electrodes electrografted with an anthraquinone diazonium compound using the rotating disc electrode method, RSC Adv., 2016, vol. 6, p. 40982.
Bousquet, A., Ceccato, M., Hinge, M., Pedersen, S.U., and Daasbjerg, K., Redox grafting of diazotated anthraquinone as a means of forming thick conducting organic films, Langmuir, 2012, vol. 28, p. 1267.
Dekanski, A., Stevanović, J., Stevanović, R., Nikolić, B.Ž., and Jovanović, V.M., Glassy carbon electrodes: I. Characterization and electrochemical activation, Carbon, 2001, vol. 39, p. 1195.
Han, S.W., Joo, S.W., Ha, T.H., Kim, Y., and Kim, K., Adsorption characteristics of anthraquinone-2-carboxylic acid on gold, J. Phys. Chem. B, 2000, vol. 104, p. 11987.
Niedziałkowski, P., Ossowski, T., Zięba, P., Cirocka, A., Rochowski, P., Pogorzelski, S.J., Ryl, J., Sobaszek, M., and Bogdanowicz, R., Poly-l-lysine-modified boron-doped diamond electrodes for the amperometric detection of nucleic acid bases, J. Electroanal. Chem., 2015, vol. 756, p. 84.
Evans, S., Correction for the effects of adventitious carbon overlayers in quantitative XPS analysis, Surf. Interface Anal., 1997, vol. 25, p. 924.
Bogdanowicz, R., Sawczak, M., Niedzialkowski, P., Zieba, P., Finke, B., Ryl, J., and Ossowski, T., Direct amination of boron-doped diamond by plasma polymerized allylamine film, Phys. Status Solidi A, 2014, vol. 211, p. 2319.
Truesdale, G.A. and Downing, A.L., Solubility of oxygen in water, Nature, 1954, vol. 173, p. 1236.
Franco, C. and Olmsted, J., Photochemical determination of the solubility of oxygen in various media, Talanta, 1990, vol. 37, p. 905.
Manisankar, P., Gomathi, A., and Velayutham, D., Oxygen reduction at the surface of glassy carbon electrodes modified with anthraquinone derivatives and dyes, J. Solid State Electrochem., 2005, vol. 9, p. 601.
Kocak, I., Ghanem, M.A., Al-Mayouf, A., Alhoshan, M., and Bartlett, P.N., A study of the modification of glassy carbon and edge and basal plane highly oriented pyrolytic graphite electrodes modified with anthraquinone using diazonium coupling and solid phase synthesis and their use for oxygen reduction, J. Electroanal. Chem., 2013, vol. 706, p. 25.
Jürmann, G., Schiffrin, D.J., and Tammeveski, K., The pH-dependence of oxygen reduction on quinone-modified glassy carbon electrodes, Electrochim. Acta, 2007, vol. 53, p. 390.
Kullapere, M. and Tammeveski, K., Oxygen electroreduction on anthraquinone-modified nickel electrodes in alkaline solution, Electrochem. Commun., 2007, vol. 9, p. 1196.
Sarapuu, A., Helstein, K., Schiffrin, D.J., and Tammeveski, K., Kinetics of oxygen reduction on quinone-modified HOPG and BDD electrodes in alkaline solution, Electrochem. Solid-State Lett., 2005, vol. 8, p. E30.
Mirkhalaf, F., Tammeveski, K., and Schiffrin, D.J., Substituent effects on the electrocatalytic reduction of oxygen on quinone-modified glassy carbon electrodes, Phys. Chem. Chem. Phys., 2004, vol. 6, p. 1321.
Vaik, K., Sarapuu, A., Tammeveski, K., Mirkhalaf, F., and Schiffrin, D.J., Oxygen reduction on phenanthrenequinone-modified glassy carbon electrodes in 0.1 M KOH, J. Electroanal. Chem., 2004, vol. 564, p. 159.
Sarapuu, A., Vaik, K., Schiffrin, D.J., and Tammeveski, K., Electrochemical reduction of oxygen on anthraquinone-modified glassy carbon electrodes in alkaline solution, J. Electroanal. Chem., 2003, vol. 541, p. 23.
Hossain, M.S., Tryk, D., and Yeager, E., The electrochemistry of graphite and modified graphite surfaces: the reduction of O2, Electrochim. Acta, 1989, vol. 34, p. 1733.
Kim, H., Goodson, T., and Zimmerman, P.M., Achieving accurate reduction potential predictions for anthraquinones in water and aprotic solvents: effects of inter- and intramolecular H-bonding and ion pairing, J. Phys. Chem. C, 2016, vol. 120, p. 22235.
Ossowski, T., Pipka, P., Liwo, A., and Jeziorek, D., Electrochemical and UV-spectrophotometric study of oxygen and superoxide anion radical interaction with anthraquinone derivatives and their radical anions, Electrochim. Acta, 2000, vol. 45, p. 3581.
ACKNOWLEDGMENTS
The DS funds of the Faculty of Electronics, Telecommunications, and Informatics of the Gdańsk University of Technology are also acknowledged.
Funding
The authors gratefully acknowledge the financial support from the Polish National Science Centre (NCN) under Grant no. 2014/14/M/ST5/00715 and the National Centre for Science and Development Grant Techmatstrateg no. 347324.
Author information
Authors and Affiliations
Corresponding authors
Rights and permissions
About this article
Cite this article
Macewicz, Ł., Skowierzak, G., Niedziałkowski, P. et al. Studies on Aminoanthraquinone-Modified Glassy Carbon Electrode: Synthesis and Electrochemical Performance toward Oxygen Reduction. Russ J Electrochem 57, 245–254 (2021). https://doi.org/10.1134/S1023193521030071
Received:
Revised:
Accepted:
Published:
Issue Date:
DOI: https://doi.org/10.1134/S1023193521030071