Abstract
Prolonged drought can negatively impact a variety of plant physiological processes. We conducted a drought experiment on one-year-old plants of Glycyrrhiza uralensis to characterize how gas exchange, chlorophyll fluorescence, the activities of antioxidant enzymes, and growth responded to droughts of varying magnitudes. Plants grown under control (no drought stress) and three droughted conditions, differing in their duration (7, 14 and 21 days). Net photosynthetic rate (Pn), transpiration rate (E), and stomatal conductance (gs) were significantly lower with increasing water stress. The moderate (14 days) and severe (21 days) water stress treatments resulted in lower Pn, as well as stomatal limitation of photosynthesis during the morning but nonstomatal limitation during the afternoon. Meanwhile, the increase in F0 and decrease in Fm, ΦPSII, and Fv/Fm further suggested that photosynthetic capacity of G. uralensis leaves was reduced and PSII partially inactivated during the day under drought. These patterns indicated that drought damaged PSII of G. uralensis leaves. However, part of the damage to PSII might be alleviated under mild or moderate drought, but it was not reparable under severe drought. Water stress decreased the Pn-PAR curves of G. uralensis and led to higher LCP and RD and lower Pmax and LSP. Together, these results suggest that drought stress weakened the light adaptability of leaves and reduced the efficiency of light transformation. In addition, the activities of antioxidant enzymes (superoxide dismutase (SOD), peroxidase (POD), and catalase (CAT)) were elevated in G. uralensis under moderate (14 days) and severe (21 days) water stress during first 14 days but were significantly reduced after that time. These results suggest that protective enzymes were activated early to resist the damage caused by drought stress but that prolonged stress caused accumulation of reactive oxygen species that exceeded the capacity of the antioxidant system, resulting in cellular damage. Water stress also reduced plant growth (lower plant height, basal diameter and crown area) and yield (smaller roots, stems, leaves, and total biomass). Moreover, water stress led to yellowing, wilting, and senescence of leaves and suppressed growth of main roots. We found that the drought treatment lasting 21 days is the moisture threshold of G. uralensis, and that longer droughts in the Hexi Corridor can cause G. uralensis to wither and die.
Similar content being viewed by others
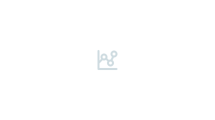
Explore related subjects
Discover the latest articles, news and stories from top researchers in related subjects.Avoid common mistakes on your manuscript.
INTRODUCTION
Global climate change is expected to increase aridity in many parts of the world. Arid and semi-arid areas account for about 40% of land area globally and more than 50% of the land area in China [1]. In arid and semi-arid areas, water stress is one of the most important abiotic factors affecting crop growth and crop yield [2]. Water stress has various impacts on plants and hinder every stage of plant growth. Severe water stress affects plant morphology, photosynthesis, growth, and metabolism, and can result in plant mortality [3, 4]. When plants experience drought stress, they can produce and accumulate reactive oxygen species (ROS) [5, 6]. Antioxidants and related enzymes are important for ROS detoxification during water stress [7]. Some of the enzymes that scavenge ROS include superoxide dismutase (SOD), catalase (CAT) and peroxidase (POD) [8]. By dismutating \({\text{O}}_{2}^{ - }\) into H2O2 and O2, SOD constitutes the only enzymatic mechanism for scavenging superoxides [9]. H2O2 can be scavenged by CAT and by POD into water and oxygen (O2). Under water stress, CAT, POD, and SOD activities increase, resulting in ROS scavenging [8]. Many plants, such as oilseed rape [10], cucumber [11], Lycium ruthenicum [7], have been shown to have physiological responses to antioxidant enzymes during water stress. However, under water stress, antioxidant enzyme activity varies among types of antioxidant and species of plants. Thus, SOD, POD, CAT and other enzymes may each respond differently to water stress depending on plant species-specific resistance to water stress.
Glycyrrhiza uralensis Fisch, a perennial herb in the family Leguminosae, is widely used in traditional Chinese medicine for various purposes such as bronchitis, ague, hepatitis, phthisis, and gastric ulcers [12]. G. uralensis also has important ecological roles in arid and semiarid regions, such as hampering wind, fixing sand, and promoting soil formation [13]. Wild G. uralensis is well adapted to growing under unfavorable conditions, such as salt and drought stress, which are widespread throughout the deserts of northwest China [14]. In the arid and semi-arid regions of Northwest China, the Hexi Corridor forms an important ecological barrier [2] where wild G. uralensis is quite abundant and is distributed mostly in the arid desert grasslands between 860 and 1500 m AMSL. Because G. uralensis is able to block wind and to stabilize sand, G. uralensis has become a very important resource in this area. However, recent over-exploitation of wild G. uralensis has led to sharp declines or even extinction of native populations [14], thus accelerating desertification of this region. As a result, commercial cultivars have become the main source for the supply market [14], helping to meet market demand while also protecting the remaining wild G. uralensis populations. Because of water shortage in the Hexi Corridor [15], water has become the most important environmental factor affecting plant growth and development. Therefore, studying the adaptability of G. uralensis to water stress may be important for successful artificial cultivation in the Hexi Corridor.
Most previous studies of G. uralensis, however, have focused on its pharmacology, and little is known about its physiological adaptations to drought. A major objective of this study, therefore, was to characterize the effects of different water conditions on G. uralensis. We grew G. uralensis under four water regimes and investigated how drought stress affected photosynthetic, chlorophyll fluorescence, and antioxidant enzymes in G. uralensis. The drought responses of G. uralensis could provide useful information to improve artificial cultivation of G. uralensis in the Hexi Corridor, potentially allowing the replacement of wild resources by artificial cultivation and alleviating stresses on wild populations.
MATERIALS AND METHODS
The study site was located at Hexi University in Zhangye City (37°28′ N, 97°20′ E), Gansu Province, China (Fig. 1). The climate in this region is continental with a mean annual rainfall of 113–120 mm and mean annual evaporation of 2291 mm. The mean annual temperature is 6°C and annual sunshine duration is 3106 h. The annual frost period lasts 138–179 days. The specific location of the study area is shown in Fig. 1.
One-year-old G. uralensis plants were selected from the Hexi Corridor Medicinal Plant Germplasm Nursery Garden of Zhangye City. Plants were of uniform size, with a root length of 15 cm. Five roots were transplanted into each plastic pot (23 cm in diameter and 20 cm in height) with the same amount of soil, sand, and humus (1 : 2 : 1) mixture on May 16, 2021. When plants had grown to an approximate height of 20 cm, we began the water stress treatment. All plastic pots were placed under a mobile rain shelter during the period of water stress in order to exclude natural rainfall. During the period from June 20 to July 11, 2021, the plants were subjected to three different watering treatments plus a control, in which plants were watered every 7 days. The three irrigation treatments consisted of irrigation intervals of 7, 14, and 21 days at a rate of 2000 mL water per pot. The timing and frequency of treatments, as well as the sampling dates (21 days) are listed in Table 1. At the end of this period, the water stress treatment was concluded and plants were harvested. Diurnal fluctuations of photosynthetically active radiation (PAR) (curve line) and air temperature (bars) for sun light from 07:00 to 19:00 on the measurement day (July 11, 2021) in Hexi University are shown in Fig. 2.
Growth measurements. At 21 day into the experiment, plant height, basal diameter, and crown area were recorded. Plant height was measured from the soil surface to the top of each plant using a ruler. Basal diameter was measured at the base of the stem using a Vernier caliper. Crown area was measured with a tape measure along two perpendicular axes. At this time, five G. uralensis seedlings of similar size were harvested from each treatment group. Each plant was separated into the roots, stems, and leaves. The samples were oven-dried for 48 h at 80°C, and their dry masses recorded. Total plant dry mass was calculated as the sum of the root, stem, and leaf dry masses.
Measurement of gas exchange parameters. Net photosynthetic rate (Pn), stomatal conductance (gs), transpiration rate (E), and intercellular CO2 concentration (Ci) and environmental parameters (Fig. 2) were measured at 7:00, 9:00, 11:00, 13:00, 15:00, 17:00, and 19:00 using a CIRAS-2 portable photosynthesis system. Measurements were taken in situ on mature, fully expanded leaves of three plants per treatment. Light response curves were measured at 2000, 1500, 1000, 500, 200, 100, 50, 20, and 0 μmol m–2 s–1 PAR under uniform conditions (30°C, 345–365 ppm (CO2 concentration in leaf chamber)). The linear regression of irradiance and Pn over a range of 0–200 μmol m–2 s–1 PAR was calculated to determine the light compensation points (LCP). The maximum net photosynthetic rate (Pmax), the light saturation point (LSP), and the dark respiration rate (RD) were estimated according to Zhang et al. [16]. All measurements were taken three times.
Chlorophyll fluorescence was measured on the same leaves as gas exchange using a pulse modulation fluorometer (FMS-2, Hansatech, UK) according to the method of Yin et al. [17]. Before chlorophyll fluorescence measurements, leaves were dark-adapted for 20 min using leaf clips. Initial fluorescence (F0) was measured with a measuring beam at a light intensity of less than 0.05 μmol m–2 s–1. Maximum fluorescence (Fm) was obtained by measuring chlorophyll fluorescence during a 2.5 s pulse of saturating light (18 000 μmol (photons) m–2 s–1). The maximum quantum yield of PSII (Fv/Fm) and the effective quantum yield of PSII (ΦPSII) were also calculated [3].
Enzyme assays. At 0, 7, 14, and 21 days, we collected plants to measure enzymatic activity. Enzymes were extracted using 0.1 g fresh plant leaves. Samples were added to liquid nitrogen and ground in 5 mL EPPS buffer (prepared by mixing 0.05 M Na phosphate buffer (pH 7.8), 1 mM ethylenediaminetetraacetic acid (EDTA), and 2% (w/v) polyvinylpyrrolidone (PVP)) at 4°C. The homogenate was centrifuged (Eppendorf 5804R, Germany) at 12 000 rpm for 20 min at 4°C, and the supernatant was prepared for analysis of the activities of superoxide dismutase (SOD), peroxidase (POD) and catalase (CAT).
SOD activity was measured by its ability to inhibit the photochemical reduction of nitroblue tetrazolium (NBT), recorded at 560 nm. The reaction mixture contained 50 mM Na phosphate buffer (pH 7.8), 33 μM NBT, 10 mM of L-methionine, 0.66 mM EDTA, and 0.0033 mM riboflavin. Reactions were conducted at 25°C under a light intensity of 1000 μmol m–2 s–1 for 20 min [7]. POD activity was determined by guaiacol coloration at 470 nm [18]. The reaction solution contained 0.1 M phosphoric acid buffer (pH 6.0), 28 μL guaiacol, 2 mM H2O2, and 20 μL of the enzyme. CAT activity was estimated by using the method of Prochazkova et al. [18]. The mixture contained 0.1 mL enzyme extracts and 2.5 mL of reaction solution (0.1 mM phosphate buffer (pH 7.0), 10 mM H2O2). A decrease in absorbance at 240 nm was used to measure CAT activity.
Statistical analyses. All experiments were conducted in a randomized complete block design replicated three or five times. All statistical analyses were performed using SPSS software (standard released version 26.0 for Windows, SPSS, USA). The least significant difference for multiple comparison tests was used to identify significant differences among all treatments at α = 0.05. Graphs were generated using Origin 8.0 software (USA).
RESULTS
Effects of Water Stress on Photosynthesis, Transpiration, Stomatal Conductance, and Intercellular CO2 Concentration
Figure 3 shows the impact of the different water stress treatments on the diurnal fluctuation of net photosynthetic rate in G. uralensis leaves (Fig. 3a). Diurnal variation of Pn of G. uralensis tended to be lower under water stress. The lowest Pn was observed in the severe (21 days) water treatment (Fig. 3a) and Pn was lower throughout the entire day except 9:00. Water stress significantly decreased stomatal conductance (gs) and E (Figs. 3b and 3d). Both gs and E had similar trends in Pn in the control treatment. Both gs and E were highest in the control treatment and displayed two daily peaks at 11:00 and 15:00 respectively. In the water stress treatments, gs and E were lower than in the control treatment on all sampling days (7, 14 and 21 days). In the water stress treatments, gs increased and reached its peak at 9:00, then decreased until 19:00, while E remained constant and low throughout the day under 7, 14 and 21 days of treatments. Under water stress, Ci exhibited a different pattern compared to other physiological parameters (Fig. 3c). Ci initially decreased and then increased after 13:00 in the control treatment (Fig. 3c). In the 7 and 14 days of water stress treatments, Ci remained low and sharply increased after 9:00 and 11:00, respectively, while in the 21 day of treatment Ci responded similar to the 14 day of treatment.
Diurnal changes in photosynthetic rate, Pn (a), stomatal conductance, gs (b), intercellular CO2 concentration, Ci (c), and transpiration rate, E (d) of G. uralensis leaves in each water stress treatment during measurement days. (1) Control, (2) 7 days, (3) 14 days, (4) 21 days of water stress treatments. Vertical bars indicate standard error of means (n = 3).
Response of Chlorophyll Fluorescence Parameters to Water Stress
Chlorophyll fluorescence parameters exhibited different responses to water stress treatments (Fig. 4). F0 remained slightly elevated at 13:00 and then decreased until 19:00 in the control treatment. With increasing water stress, F0 also increased. The highest F0 occurred in the 14 and 21 days of water stress treatments (Fig. 4a). F0 was significantly higher at 15:00 and then decreased until 19:00 in the 14 and 21 days of treatments. The values of Fm, Fv/Fm and ΦPSII displayed similar trends (Figs. 4b, 4c), decreasing at first and then reaching their minima between 11:00 and 15:00 under different water stress treatments. Fm, Fv/Fm and ΦPSII were highest in the control treatment, while in the 21 day of treatment these parameters were the lowest (Figs. 4b–4d). Meanwhile Fv/Fm and ΦPSII recovered quickly in the control treatment, even to higher than their initial starting values, but in the 21 day of water stress treatment they did not recover (Figs. 4c, 4d).
Diurnal changes in chlorophyll fluorescence parameters: minimal fluorescence, F0 (a), maximum fluorescence, Fm (b), maximum PSII photochemical efficiency, Fv/Fm (c), and the quantum yield of PSII (ΦPSII) (d), in leaves of G. uralensis under different water stress treatments during measurement days. (1) Control, (2) 7 days, (3) 14 days, (4) 21 days of water stress treatments. Vertical bars indicate standard error of means (n = 3).
P n-PAR Response Curves
Water stress also affected light response curves (Fig. 5): Pn was lower with increasing water stress. When PAR was higher than 500 μmol m–2 s–1, Pn was significantly lower in the water stress treatments (7, 14 and 21 days) than in the control treatment (P < 0.05). Pn increased until about 1500 μmol m–2 s–1 and then declined slightly in the control plants, but in the 14 and 21 days of water stress treatments, the peak Pn occurred at about 500 μmol m–2 s–1 (Fig. 5). Water stress also significantly influenced Pmax. Pmax decreased with increasing water stress, and the lowest value was observed under the 21 days of treatment. The LSP, LCP and RD were also affected by water stress treatments (Table 2). Under the different water stress treatments, LSP decreased sharply with increasing water stress, while the LCP and RD showed increased with increasing water stress.
Effects of Water Stress on SOD, POD, and CAT Activity
Figure 6 shows the effects of water stress on the enzymatic activities SOD, POD, and CAT in G. uralensis. The three enzymes differed in their responses to water stress (Fig. 6). The control treatment and 7 days of treatment had similar pattern, causing increased SOD during the 21 days of water stress treatment period (Fig. 6a). Meanwhile POD and CAT activities remained constant compared to the control treatment in the mild (7 days) water stress treatment (Figs. 6b, 6c). The changes in SOD, POD, and CAT enzyme activities under moderate (14 days) and severe (21 days) water stress treatments were similar (Figs. 6a–6c). Activities of all three enzymes gradually increased until their maxima at about 14 days in the moderate (14 days) and severe (21 days) water stress treatments, then decreased until the drought was over (Figs. 6a–6c). During first 14 days, activities of the three enzymes in the severe (21 days) treatment were even higher than those of the other three treatments. However, the severe (21 days) treatment showed significantly decreased SOD, POD, and CAT activities after 21 days compared to the control treatment (Figs. 6a–6c).
Response of Morphological Characteristics to Water Stress
Growth parameters exhibited significant responses to water stress treatments (Table 3). Plant height, basal diameter, and crown area were significantly smaller in the water stress treatments than in the control treatment. The severe (21 days) water stress treatment resulted in the smallest plants, with plant height, basal diameter, and crown area being 42, 38, and 68% lower, respectively, than the control treatment. Dry matter allocation was also altered significantly in the water stress treatments (Table 3). Compared to the control treatment, all dry matter parameters (root, stem, leaf, and whole plant) were significantly smaller under water stress. Dry masses were lowest for the severe (21 days) treatment, followed by the 14 and 7 days of treatments. In the 21 day of treatment, root, stem, leaf, and whole plant dry matter contents were reduced by 75, 69, 85, and 77%, respectively, compared to the control treatment.
Effect of water stress on the growth of G. uralensis can also be confirmed by Fig. 7. Under normal water supply conditions (control treatment), G. uralensis grew well, with dark green leaves and developed root systems, characterized by strong taproots and well-developed lateral roots (Fig. 3). After 7 days of water stress, the leaves remained dark green and slightly curled, but the leaves and lateral roots were significantly smaller/fewer in number compared to the control treatment. After 14 days of water stress, some leaves had turned yellow and showed signs of wilting, and a large number of lateral roots had dried up and died. After 21 days of water stress, the leaf edges were curled, withered, and necrotic, and the taproots were dwarfed and lacking obvious lateral roots (Fig. 7).
DISCUSSION
Drought is the main environmental factor limiting plant development, photosynthesis, growth, and yield, and droughts are becoming increasingly severe in arid regions due to changes in global climate [19, 20]. Photosynthesis is exceptionally sensitive to water stress because drought can cause stomatal closure and reduce mesophyll conductance, thereby limiting CO2 uptake [2]. When plants suffer prolonged, severe droughts, Pn, E, and gs are significantly lower, resulting in lower growth and even death [21]. Our results were consistent with this general pattern. We found that water stress reduced Pn and gs of G. uralensis (Figs. 3a, 3b). Lower Pn was observed in both the moderate (14 days) and severe (21 days) water tress treatments (Fig. 3a), and Pn was lower throughout the whole day except for the early morning (9:00), indicating that drought conditions might intensify reduced photosynthetic capacity and photoinhibition during the day. Reductions in E corresponded with reduced Pn and gs (Figs. 3a, 3b, 3d). This reduction in water loss driven by stomatal closure can enhance water use efficiency as drought develops [22]. During severe water stress, stomatal closure in G. uralensis conserves water, typical of most mesophytes. Although water stress significantly reduced Pn, gs, and E of G. uralensis (Figs. 3a, 3b, 3d), diurnal variation of Ci showed a different pattern. Ci initially declined and then increased at 13:00 in the control treatment (Fig. 3c), but under moderate (14 days) and severe (21 days) water stress treatments, Ci remained low and sharply increased at 11:00. Reductions in Pn and gs under moderate (14 days) and severe (21 days) water stress were accompanied by reductions in Ci before 11:00. However, under moderate (14 days) and severe (21 days) water stress reductions in Pn and gs were accompanied by relatively high Ci after 11:00. According to Farquhar and Sharkey, the former would indicate that under water stress stomatal limitation to photosynthesis was dominating in the morning of G. uralensis [15]. The latter suggested that nonstomatal limitation was dominating in the afternoon. Our data agree with previous findings reported for other species, such as common reed [15] and Sophora davidii seedlings [20].
However, gas exchange measurements do not completely characterize the effects of abiotic stress on plant processes [20]. When leaves are subjected to water stress in the light, they can be damaged by photoinhibition [12]. Analysis of chlorophyll fluorescence is becoming an increasingly popular technique for detecting the functional status. Of the photosynthetic system [11]. Chlorophyll fluorescence parameters such as F0, Fm, Fv/Fm, and ΦPSII are widely used [3]. F0 represent the fluorescence yield when the reaction center of photosystem II (PSII) is fully open [15]. We found that F0 of G. uralensis leaves increased with increasing water stress, with the highest F0 values occurring in the moderate (14 days) and severe (21 days) water stress treatments (Fig. 4a). This suggested that PSII damage/inactivation occurred when G. uralensis was subjected to severe drought stress. Similar results have been reported for sugar beet seedlings [23] and common reed [15]. The Fm value reflects the electron transfer status of PSII, while ΦPSII is positively correlated with PSII activities and can be used to evaluate the electron transfer rate of PSII [15]. We found that Fm and ΦPSII of G. uralensis leaves were significantly lower under drought stress (Figs. 4b, 4d), indicating that the electron transfer rate of the PSII reaction center was significantly suppressed by drought [15], similar to results seen in sugar beet seedlings [23]. Fv/Fm is a measure of the maximum photochemical efficiency of PSII when all the reaction centers are open [15]. When plants are not under stress, Fv/Fm is generally above 0.8, with values in the range of 0.80–0.85 indicating a healthy physiological state [24]. When plants suffer severe drought stress, Fv/Fm declines [3]. Our results were consistent with these general trends, as increasing drought stress caused Fv/Fm to decline sharply until about 0.69 (Fig. 4c), indicating that under severe (21 days) water stress PSII was inhibited. Finally, the photosynthetic capacity of G. uralensis leaves was also suppressed by drought stress [15]. However, the values of Fv/Fm and ΦPSII were the lowest and could not recover under severe (21 days) water stress (Figs. 4c, 4d), while milder water stress treatments allowed for faster recovery, which typically occurred within the day. The rate of PSII recovery in these milder water stress treatments was almost the same as in the beginning of the day. These results suggest that plants can recover rapidly from mild droughts, but they are unable to recovery from severe droughts. The damaged photosynthetic apparatus of PSII could not recover within a relatively short time and further lost its capability to dissipate heat under water stress [3].
Most studies have shown that environmental stresses, such as drought, low temperature, low light intensity, and elevated CO2 concentrations have significant effects on Pn-PAR response parameters, such as Pmax, AQY, LCP, LSP, RD [25]. Changes in the maximum net photosynthetic rate (Pmax), light compensation point (LCP), and light saturation point (LSP) of plant leaves are the main photosynthetic responses to adverse conditions [26]. Normally, Pmax, which determines the potential photosynthetic capacity, is proportional to the number of active catalytic sites in chloroplasts that are involved in the reductive assimilation of CO2 [20]. The LCP and the LSP are the two main indicators of the light requirements of the plant [27]. Our Pn-PAR curves (Fig. 5) indicated that Pmax and LSP of G. uralensis seedlings declined while the LCP increased with increasing water stress, consistent with reports on other species such as Cyclobalanopsis glauca [28] and P. euphratica [29] (Table 2). Our results confirmed that water stress reduced the photosynthetic ability of G. uralensis leaves, narrowed the range of light intensities that could be utilized, and reduced its adaptability to the light environment [15]. RD plays an important role in carbon sequestration for individual plants, plant communities, and even ecosystems, and it is sensitive to water stress [30]. In this study, increasing water stress significantly increased RD of G. uralensis plants (Table 2). The increase in RD resulted in more CO2 release at night. Therefore, a decrease in assimilation caused by water stress during the day and an increase in respiration at night could be the main drivers of reduced plant growth and productivity [21].
Plants can produce reactive oxygen species (ROS) under normal and adverse conditions [5]. In general, the production and removal of reactive oxygen species are in a dynamic balance, so that ROS will not cause excessive damage to plant cells. However, when plants experience stress, ROS can accumulate, thereby damaging plant cells [14, 31]. SOD, CAT and POD are important free-radical scavenging enzymes in plants. SOD converts oxygen free radicals (\({\text{O}}_{2}^{ - }\)) produced during stress into hydrogen peroxide (H2O2), and POD and CAT further convert H2O2 into water (H2O) and oxygen (O2) [32]. In our study, increasing drought stress led to rapid increases in SOD, POD, and CAT enzyme activities, that were then followed by a decline in their activity (Figs. 6a–6c). At about 14 days, the SOD, POD, and CAT enzyme activities in the severe (21 days) water stress treatment were even higher than those in the other three water treatments, but they declined significantly after this time point (Figs. 6a–6c). The reason may be that in the early stages of water stress, protective enzymes were activated to resist the damage caused by the adverse environment, indicating mechanism of self-regulation and adaptation. With increasing water stress, the activity and balance of the protective enzyme system were destroyed, leading to excessive accumulation of ROS. When ROS levels exceeded capacity of the antioxidant system, cellular components are damaged [7]. Our results showed that enhancement of SOD, POD, and CAT enzyme activities in G. uralensis leaves reduced the effects of drought on G. uralensis leaves during the initial stages of stress, but they could not prevent damage caused by persistent drought. These results were similar to those reported for Brassica napus L. [33] and Lycium ruthenicum Murr [7].
Drought is the most important abiotic stress factor limiting plant growth and productivity [34]. Drought causes the significant changes in growth, chlorophyll content, water content, and fluorescence parameters [34]. Many studies have found that prolonged drought can limit plant growth and biomass production, alter patterns of biomass allocation, and even cause plant death [35]. The present study showed that water stress not only reduced growth parameters of G. uralensis, such as plant height, basal diameter, and crown area, but drought also reduced biomass of G. uralensis. For example, drought led to lower dry mass of roots, stems, and leaves (Table 3). Increasing water stress also caused leaves of G. uralensis to turn from green to yellow and caused leaf margins to curl, ultimately leading to wilting and desiccation (Fig. 7). Water stress also suppressed growth of the main root, led to fewer lateral roots, and caused the root system to dry (Fig. 7). All of these effects are thought to be due to water stress causing damage to the efficiency of PSII, resulting in photoinhibition. These results for G. uralensis in the Hexi Corridor are similar to those of Oriental Lily in the Horqin Sandy Land [21]. The higher photosynthetic parameters better growth in the control treatment indicate that if water is not restricted, then G. uralensis can grow well in the Hexi Corridor.
In conclusion, water stress significantly affected leaf CO2 uptake and photosynthetic biochemistry in G. uralensis. Water stress limited photosynthesis not only by decreasing Pn, gs, E, Pmax, and LSP, but also by increasing Ci, RD and LCP. These results suggest that as stress becomes more severe, both stomatal and non-stomatal factors restrict photosynthesis. Stomatal limitation dominated in the morning, and nonstomatal limitation dominated in the afternoon under moderate and severe water stress treatments. Meanwhile water stress weakened light adaptability and reduced the potential photosynthetic capacity of G. uralensis leaves. This was consistent with increases in F0 and decreases in Fm, ΦPSII, and Fv/Fm, which indicated that the photosynthetic capacity of G. uralensis leaves was reduced, that PSII was partially inactivated, and that the photosynthetic apparatus of PSII was destroyed. However, some of these adverse effects on PSII might be recovered under mild or moderate drought but not under severe water stress. The SOD, POD, and CAT activities were initially elevated but then significantly declined under moderate and severe water stress, indicating that plants can initially resist water stress by activating enzymes, but when drought exceeds a certain threshold the ability of cells to eliminate ROS was reduced, leading to cellular damage. Drought stress eventually led to reduced plant growth (lower plant height, basal diameter, and crown area) and yield (smaller roots, stems, leaves, and total dry mass) of G. uralensis under water stress. Thus, under high light intensity in the Hexi Corridor, 21 days of continuous drought is a threshold after which G. uralensis cannot ecover. If drought exceeds 21 days, the G. uralensis plants will wither and even die.
REFERENCES
Jiang, Z.Q., Li, C.Z., Yu, L.E., and Tang, G.G., Effects of drought stress on MDA content and 3 antioxidant enzymes activities in leaves of Carya illinoensis, Acta Agriculturae Shanghai, 2019, vol. 35(1), p. 7.
Zhang, Y.J., Li, Y.H., Gao, H., Wang, H.L., Sheng, K.D., Guo, Y.Y., Yan, F., Lu, K., Tian, J.W., and Lu, Y.L., Effect of drought stress on leaf gas exchange, chlorophyll content and dry matter allocation of Phragmites australis in the Heihe River Basin, Philipp. Agric. Scientist, 2019, vol. 102(2), p. 141.
Guo, Y.Y., Yu, H.Y., Kong, D.S., Yan, F., and Zhang, Y.J., Effects of drought stress on growth and chlorophyll fluorescence of Lycium ruthenicum Murr. seedling, Photosynthetica, 2016, vol. 54(4), p. 524.
Rodiyati, A., Arisoesilaningsih, E., Isagi, Y., and Nakagoshi, N., Responses of Cyperus brevifolius (Rottb.) Hassk. and Cyperus kyllingia Endl. to varying soil water availability, Environ. Exp. Bot., 2005, vol. 53, p. 259.
Ozgur, R., Uzilday, B., Sekmen, A.H., and Turkan, I., Reactive oxygen species regulation and antioxidant defense in halophytes, Funct. Plant Biol., 2013, vol. 40, p. 643.
Lima, J.V. and Lobato, A.K.S., Brassinosteroids improve photosystem II efficiency, gas exchange, antioxidant enzymes and growth of cowpea plants exposed to water deficit, Physiol. Mol. Biol. Plants, 2017, vol. 23, p. 59.
Guo, Y.Y., Yu, H.Y., Yang, M.M., Kong, D.S., and Zhang, Y.J., Effect of drought stress on lipid peroxidation, osmotic adjustment and antioxidant enzyme activity of leaves and roots of Lycium ruthenicum Murr. seedling, Russ. J. Plant Physiol., 2018, vol. 65, p. 244.
Mittler, R., Vanderauwera, S., Gollery, M., and Van Breusegem, F., The reactive oxygen gene network of plants, Trends Plant Sci., 2004, vol. 9, p. 490.
Zhou, Y.H., Yu. J.Q., Huang. L.F., and Nogués. S., The relationship between CO2 assimilation, photosynthetic electron transport and water-water cycle in chill-exposed cucumber leaves under low light and subsequent recovery, Plant Cell Environ., 2004, vol. 27, p. 1503.
Liu, D., Wu, L.T., Naeem, M.S., Liu, H.B., Deng, X.Q., Xu, L., Zhang, F., and Zhou, W.J., 5-Aminolevulinic acid enhances photosynthetic gas exchange, chlorophyll fluorescence and antioxidant system in oilseed rapeunder drought stress, Acta Physiol. Plant., 2013, vol. 35, p. 2747.
Fan, H.F., Ding, L., Xu, Y.L., and Du, C.X., Antioxidant system and photosynthetic characteristics responses to short-term PEG-induced drought stress in cucumber seedling leaves, Russ. J. Plant Physiol., 2017, vol. 64(2), p. 162.
Liao, J.X. and Wang, G.X., Effects of drought stress on leaf gas exchange and chlorophyll fluorescence of Glycyrrhiza uralensis, Russ. J. Ecol., 2014, vol. 45(6), p. 532.
Zhang, W.J., Xie, Z.C., Zhang, X.J., Lang, D.Y., Zhang, X.H., Growth-promoting bacteria alleviates drought stress of G. uralensis through improving photosynthesis characteristics and water status, J. Plant Interact., 2019, vol. 14(1), p. 580.
Zhang, W.J., Xie, Z.C., Wang, L.H., Li, M., Lang, D.Y., Zhang, X.H., Silicon alleviates salt and drought stress of Glycyrrhiza uralensis seedling by altering antioxidant metabolism and osmotic adjustment, J. Plant Res., 2017, vol. 130, p. 611.
Zhang, Y.J., Gao, H., Li, Y.H., Wang, L., Sheng, K.D., Guo, Y.Y., Yan, F., Wang, Y.W., Lu, K., Tian, J.W., and Lu, Y.L., Effect of water stress on photosynthesis, chlorophyll fluorescence parameters and water use efficiency of common reed in the Hexi Corridor, Russ. J. Plant Physiol., 2019, vol. 66, p. 556.
Zhang, Y.J., Yan, F., Gao, H., Xu, Y.Z., Guo, Y.Y., Wang, E.J., Li, Y.H., and Xie, Z.K., Chlorophyll content, leaf gas exchange and growth of oriental lily as affected by shading, Russ. J. Plant Physiol., 2015, vol. 62, p. 334.
Yin, C.Y., Wang, X., Duan, B.L., Luo, J.X., and Li, C.Y., Early growth, dry matter allocation and water use efficiency of two Sympatric populus species as affected by water stress, Environ. Exp. Bot., 2005, vol. 53, p. 315.
Prochazkova, D., Sairam, R.K., Srivastava, G.C., and Singh, D.V., Oxidative stress and antioxidant activity as the basis of senescence in maize leaves, Plant Sci., 2001, vol. 161, p. 765.
Yuan, X.K., Yang, Z.Q., Li, Y.X., Liu, Q., and Han, W., Effects of different levels of water stress on leaf photosynthetic characteristics and antioxidant enzyme activities of greenhouse tomato, Photosynthetica, 2016, vol. 54, p. 28.
Wu, F.Z., Bao, W.K., Li, F.L., and Wu, N., Effects of water stress and nitrogen supply on leaf gas exchange and fluorescence parameters of Sophora davidii seedlings, Photosynthetica, 2008, vol. 46, p. 40.
Zhang, Y.J., Xie, Z.K., Wang, Y.J., Su, P.X., An, L.P., and Gao, H., Effect of water stress on leaf photosynthesis, chlorophyll content, and growth of oriental lily, Russ. J. Plant Physiol., 2011, vol. 58, p. 844.
Ramanjulu, S., Sreenivasalu, N., Giridhara Kumar, S., and Sudhakar, C., Photosynthetic characteristics in mulberry during water stress and rewatering, Photosynthetica, 1998, vol. 35, p. 259.
Li, G.L., Wu, H.X., Sun, Y.Q, and Zhang, S.Y., Response of chlorophyll fluorescence parameters to drought stress in sugar beet seedlings, Russ. J. Plant Physiol., 2013, vol. 60, p. 337.
Johnson, G.N., Young, A.J., Scholes, J.D., and Horton, P., The dissipation of excess excitation energy in British plant species, Plant, Cell Environ., 1993, vol. 16, p. 673.
Feng, Z.Z., Wang, X.K., Zheng, Q.W., Feng Z.W., Xie J.Q., and Chen, Z., Response of gas exchange of rape to ozone concentration and exposure regime, Acta. Ecol. Sinica, 2006, vol. 26(3), p. 823.
Gao, Y., Xia, J.B., Chen, Y.P., Zhao, Y.Y., Kong, Q.X., and Lang, Y., Effects of extreme soil water stress on photosynthetic efficiency and water consumption characteristics of Tamarix chinensis in China’s Yellow River Delta, J. For. Res., 2017, vol. 28(3), p. 491.
Zhao, J., Yang, R., Liu, X.H., Zhang, G.C., and Zhang, S.Y., How soil moisture affects photosynthetic characteristics of Aralia elata leaves, Pol. J. Environ. Stud., 2018, vol. 27(4), p. 1869.
Wei, L.Y., Huang, Y.Q., Li, X.K., Mo, L., and Yuan, W.Y., Effects of soil water on photosynthetic characteristics and leaf traits of Cyclobalanopsis glauca seedlings growing under nutrient rich and poor soil, Acta. Ecol. Sinica, 2009, vol. 29, p. 160.
Li, J.Y., Zhao, C.Y., Li, J., Yan, Y.Y., Yu, B., and Han, M., Growth and leaf gas exchange in Populus euphratica across soil water and salinity gradients, Photosynthetica, 2013, vol. 51(3), p. 321.
Xu, W.Z., Deng, X.P., and Xu, B.C., Effects of water stress and fertilization on leaf gas exchange and photosynthetic light-response curves of Bothriochloa ischaemum L., Photosynthetica, 2013, vol. 51(4), p. 603.
Li, W.M., Wang, Y.J, Wei, H.L., Zhang, Y.B., Guo, Z.H., Qiu, Yang., Wen, L.R., and Xie, Z.K., Structural characterization of Lanzhou lily (Lilium davidii var. unicolor) polysaccharides and determination of their associated antioxidant activity, J. Sci. Food Agric., 2020, p. 1.
Guo, Y.Y., Zhang, W.H., He, J.F., Zhou, J.Y., and Yu, H.Y., Effect of water stress and seed mass on germination and antioxidative enzymes of Xanthoceras sorbifolia, Afr. J. Biotechnol., 2012, vol. 18, p. 4187.
Mirzaee, M., Moieni, A., and Ghanati, F., Effects of drought stress on the lipid peroxidation and antioxidant enzyme activities in two canola (Brassica napus L.) cultivars, J. Agric. Sci. Technol., 2013, vol. 15, p. 593.
Ahmad, Z., Anjum, S., Waraich, E.A., Ayub, M.A., Ahmad, T., Tariq, R.M. S., Ahmad, R., and Iqbal, M.A., Growth, physiology, and biochemical activities of plant responses with foliar potassium application under drought stress – A review, J. Plant Nutr., 2018, vol. 41, p. 1734.
Li, F.L., Bao, W.K., and Wu, N., Effects of water stress on growth, dry matter allocation and water-use efficiency of a leguminous species, Sophora davidii, Agroforest Syst., 2009, vol. 77, p. 193.
ACKNOWLEDGMENTS
I would like also to thank my daughter Zixuan Gao and I love you forever.
Funding
This study was funded by the Project of Science and Technology Plan of Gansu Province (21JR7RA555), the Project of Key Laboratory of Hexi Corridor Characteristic Resources Utilization of Gansu Province (XZ2004), the project of Higher Education Industry Support and Guidance of Gansu Province (2019A-01), the project of Higher Education Innovation Ability Promotion of Gansu Province (2019B-143) and the project of Longyuan Innovation and Entrepreneurship Talent (Team) of Gansu Province.
Author information
Authors and Affiliations
Corresponding author
Ethics declarations
The authors declare that they have no conflicts of interest.
This article does not contain any studies involving animals or human participants as objects of research.
Rights and permissions
About this article
Cite this article
Gao, H., Bai, N., Zhang, Y. et al. Drought Stress Alters Gas Exchange, Chlorophyll Fluorescence, and Antioxidant Enzyme Activities in Glycyrrhiza uralensis in the Hexi Corridor, China. Russ J Plant Physiol 69, 123 (2022). https://doi.org/10.1134/S102144372206005X
Received:
Revised:
Accepted:
Published:
DOI: https://doi.org/10.1134/S102144372206005X