Abstract
While brain size in primates and their relatives within Euarchontoglires is well-studied, less research has examined brain shape, or the allometric trajectories that underlie the relationship between size and shape. Defining these patterns is key to understanding evolutionary trends. 3D geometric morphometric analyses of endocranial shape were performed on 140 species of extant euarchontoglirans using digital cranial endocasts. Principal component analyses on Procrustes shape variables show a clear phylogenetic pattern in endocranial shape, supported by an ANOVA which identified significant differences in shape among several groups (e.g., Platyrrhini, Strepsirrhini, Scandentia, Rodentia, and Lagomorpha). ANOVAs of shape and size also indicate that allometry has a small but significant impact on endocranial shape across Euarchontoglires, with homogeneity of slopes tests finding significant differences in the scaling relationship between shape and size among these same groups. While most of these clades possess a distinct endocranial morphotype, the highly derived platyrrhines display the strongest relationship between size and shape. Rodents show the most diversity in endocranial shape, potentially attributed to their comparatively weak relationship between shape and size. These results suggest fundamental differences in how shape and size covary among Euarchontoglires, which may have facilitated the adaptive radiations that characterize members of this group.
Similar content being viewed by others
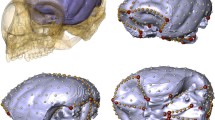
Introduction
Allometry refers to patterns of variation between a trait and body size and the covariance among traits as body size changes1. As such, understanding allometric patterns is fundamental to the study of morphological diversification. Despite its role in diversification, the nature and extent to which allometry impacts morphology can be difficult to assess as it is a product of the dynamic influence of multiple factors2,3 which can be expressed differently during growth and development (ontogenetic allometry), among individuals of the same age group within a species (static allometry), and among species or clades (evolutionary allometry4).
The relationship between body size and overall brain size is well established within Mammalia5. Unsurprisingly, species with larger bodies tend to have larger brains than smaller species. However, the slope of this relationship is highly variable across Mammalia, a feature which suggests a relaxation of allometric influence on brain size relative to non-avian vertebrates5,6,7. Importantly, the brains of larger species are not necessarily scaled up versions of the same design seen in smaller species, emphasizing the importance of considering more than just size in studying allometry. For instance, the relatively large brains of primates are attributed to a disproportionate expansion of the neocortex, which constitutes as much as 80% of brain volume in anthropoids8. This implies either a weak allometric constraint on brain morphology or that different regions of the brain may be impacted by allometry to varying degrees across lineages.
Recent analyses have examined allometry of brain shape using landmark based geometric morphometrics9. However, the challenges of developing landmark sets applicable to groups with significant morphological diversity has meant that these analyses tend to be phylogenetically restricted (e.g., to hominoids10,11; to Primates9) and therefore may be missing the broader evolutionary context from which unique trajectories emerged. Given the distinct allometries identified in brain size among major mammalian clades5,6,7,12, as well as the distinct allometries identified in shape within some groups (i.e., Primates9), it could be predicted that allometry will impact shape differently among major clades. However, this hypothesis has not been directly tested.
In this study, we use a set of rigorously validated landmarks13 developed to capture variation in major brain regions (i.e., neocortex, paleocortex, cerebellum [and petrosal lobules], brainstem, and olfactory bulbs) among groups with significant diversity in endocranial shape. Using these landmark data, we investigate the impact of evolutionary allometry on brain shape among major clades of Euarchontoglires, the Supraorder which includes Scandentia, Dermoptera, Primates, Rodentia, and Lagomorpha. Specifically, the goal of this analysis is to (1) describe endocranial shape variation among major clades of Euarchontoglires and (2) assess the impact of evolutionary allometry on endocranial shape among these groups. Allometry is a significant factor influencing morphological diversification, and as a result, investigation into this process is integral to understanding the evolution of biological variation. The detailed examination of allometry provided here will help to establish a more holistic view of brain evolution within this group which will help to frame potential adaptive interpretations of morphological variation in the Euarchontoglires brain.
Results
For the PCA of endocranial shape within this sample of extant Euarchontoglires, the broken stick model indicates that the PCs comprising most of the significant variation are limited to the first four dimensions (Fig. S1), representing approximately 72.9% of variation in endocranial shape. In the PCA (Fig. 1) there is clear separation between many clades across the morphospace. The phylogenetic signal for shape (K-mult = 0.68) and size (K-mult = 0.66) for the full dataset is less than expected under Brownian motion. Phylogenetic signals for shape and size also vary among clades (Table 1). Platyrrhines have the strongest phylogenetic signal (K-mult = 0.80) in endocranial shape while lagomorphs have the weakest signal (K-mult = 0.28). Relative to shape, there is a stronger phylogenetic signal in endocranial size for all clades (Table 1). Though the phylogenetic signal in size for most of these clades is less than expected under Brownian motion, in the case of the platyrrhines and lagomorphs the phylogenetic signal exceeds the Brownian motion model.
(A) PCA of Procrustes coordinates for seven major clades of Euarchontoglires. Surface warps of PC extremes are based on the mean species in the entire sample (Paraxerus cepapi [USNM 367956]) representing PC1 and PC2 maximum and minimum and scaled to maximum endocranial length. (B) PCA showing PCs 3 and 4. Hollow diamonds represent Lorisiformes, filled diamonds represent Lemuriformes. Hollow squares represent Ochotonidae, filled squares represent Leporidae.
The ANOVA of endocranial shape and clade suggests that ~ 58% (p = 0.0001; Table S1) of the variation in endocranial shape can be attributed to clade membership. Significant differences in endocranial shape were identified among all clades. Given the distinct morphospace occupied by ochotonids and leporids, an additional ANOVA was run with these two clades separated. The results of this test found that the difference in shape between these clades was near, but not within, the significance threshold of α = 0.05 (Z = 1.52, p = 0.071; Table S3). This may be due to the low sample size reducing statistical power.
Surface deformations indicate that PC 1 is capturing variation in the scale of the neocortex such that species occupying the positive extreme of this axis (e.g. Platyrrhini) have large neocortices both mediolaterally and ventrodorsally, with the neocortex covering the dorsal aspect of the cerebellum, whereas species at the negative extreme of this axis (i.e. Rodentia) have a small, shallow neocortex, which does not entirely cover the dorsal aspect of the cerebellum with a paleocortex that accounts for more of the visible cerebral cortex. PC 1 also appears to capture variation in the scale of the olfactory bulbs and the flexion of the endocast, particularly in its ventral aspect. Species which occur on the negative aspect of PC 1 possess a larger olfactory bulb relative to the neocortex and an unflexed ventral surface, such that the olfactory bulbs are the most rostral structure, and the brainstem is the most caudal structure. Alternatively, species at the positive aspect of PC 1 possess relatively small olfactory bulbs which are nearly level with the neocortex at the rostral end of the endocast, and a brainstem which exits more ventrally such that the cerebellum is the most dorsal structure. For dorsal views of the surface warps associated with PCs 1 and 2 see Fig. S2.
There is less separation in endocranial shape across PC 2, which appears to primarily reflect the rostro-dorsal compactness of the endocast overall. Species that occur on the positive aspect of this PC (e.g., Strepsirrhini, Scandentia, and Rodentia) have a ventrodorsally deep and rostrocaudally compressed endocast while species on the negative aspect of this PC (e.g., Lagomorpha) have a shallower endocast ventrodorsally which is relatively more elongated rostrocaudally. Additionally, this PC captures variation in the constriction of the circular fissure (which separates the olfactory bulbs from the rest of the endocast), and in the flexion of the rostral aspect of the paleocortex. Species which occur on the positive aspect of this PC possess a ventrodorsally deep and mediolaterally wide circular fissure and a correspondingly large olfactory bulb relative to species which occur on the negative aspect, which possess a more constricted circular fissure with olfactory bulbs and rostral paleocortex which are flexed ventrally.
The separation across PC 1 may reflect allometry, as typically larger bodied haplorhines occur on the far positive axis and the typically smaller bodied rodents occur on the negative extreme of this PC. The ANCOVAs of endocranial shape, size, and clade indicate that a small but significant portion of the variation in endocranial shape is explained by changes in size (Table 2). Significantly, there appears to be an interaction between centroid size and clade, which suggests there may be distinct allometric scaling relationships among these clades. This is corroborated by the homogeneity of slopes tests which supports the unique allometry models over the common allometry models in the phylogenetic (p = 0.047) and non-phylogenetic models (p = 0.016) tests (S4 and S7), though the amount of variance explain by these parameters is low (R2 = 0.06 and 0.031 respectively). Regardless, this means that shape changes differently with size among these clades and therefore allometry cannot be corrected for. Subsequent pairwise tests of means, vector lengths, and angles are reported in Supplementary Information (Tables S4–S9). Within the non-phylogenetic model, significant differences in means are found among all combinations of platyrrhines, strepsirrhines, lagomorphs, and rodents. Significant differences in vector lengths and angles are observed between several clades. Most notably, lagomorphs are significantly different from most clades, including rodents, in both angle and vector length. Though platyrrhines and strepsirrhines differ in allometric means they do not differ significantly in vector length or angle in the non-phylogenetic model. Within the phylogenetic model, significant differences in allometric means are found only between platyrrhines vs. rodents and strepsirrhines. Similar to the non-phylogenetic tests, lagomorphs are distinct from several other clades, including rodents, in both vector length and angle, and platyrrhines and strepsirrhines do not significantly differ from each other in either vector length or angle.
Figure 2a shows regression scores of endocranial shape fitted against log centroid size. There is no overlap between primates and any other order in regression score, which suggests that there is no overlap in shape for species of the same size between primates and other euarchontoglirans. Figure 2b shows the distinct shape size scaling relationships in PC1 across these orders. Rodents show a relatively shallow allometric slope with little change in shape across PC 1 associated with changes in size. This is corroborated by the low impact of size on shape for the order overall (Table 1). Size has a similar impact on shape across Scandentia, Platyrrhini and Strepsirrhini, though the relationship is no longer significant when phylogeny is controlled for (Table 1). A similar pattern is seen in lagomorphs as the impact of size on shape within the linear model is high (R2 = 0.416); this can also be seen in the steep slope shown Fig. 2b, but substantially decreases within the phylogenetic model where the relationship is no longer statistically significant. Despite their close relationship platyrrhines and strepsirrhines appear to have inverse size scaling relationships for shape values across PC 1, with the latter having a more similar slope to the scandentians.
(A) regression scores of endocranial shape against log centroid size. The grey dashed line represents separation between primates and all other clades. (B) fitted values based on PC1 shape data against log centroid size. (C–G) Lateral view of wireframe warps representing changes in shape associated with changes in size for each clade based on clade-specific regressions scores of endocranial shape fitted against log centroid size. Red represents the shape associated with maximum size and blue represents the shape associated with minimum size. Dashed lines represent wireframe connections on the ventral surface of the endocast, dotted lines represent wireframe connections on the lateral surface of the endocast. (C) Platyrrhini, (D) Strepsirrhini, (E) Scandentia, (F) Rodentia, (G) Lagomorpha. Wireframes are not derived from the trajectory shown in (A).
Wireframes produced from standardized shape scores plotted against size show how allometry impacts endocranial shape differently within each clade (lateral views shown in Fig. 2C–G; dorsal views shown in Fig. S3). Within Platyrrhini, increased size (centroid size) is associated with a dorsally expanded neocortex as well as proportionally smaller olfactory bulbs. Increased size in Strepsirrhini is also associated with a smaller olfactory bulb, as well as a ventrally expanded neocortex and paleocortex, and a laterally narrower neocortex and cerebellum. Size related changes in Scandentia are subtler, but increased size appears to be associated with a ventro-dorsally shallower neocortex and a slightly shallower paleocortex. Increased size in Rodentia is associated with a decreased diameter of the circular fissure and a shallower paleocortex and rostral aspect of the brain stem. Finally, increased size in Lagomorpha is associated with a ventro-dorsally deeper neocortex, a rostro-caudally shorter cerebellum, and smaller petrosal lobules. To see how the wireframe connections relate to the anatomical brain regions see Fig. S4.
Discussion
At the ordinal level, phylogeny appears to have a strong influence on endocranial shape. This is apparent by the dispersion of these orders across the shape space, which shows separation among several clades (Fig. 1) and is supported by the ANOVA, which found significant differences in endocranial shape among all clades (Dermoptera and Tarsiidae not included). This is surprising considering that the phylogenetic signal in endocranial shape across this entire dataset (K = 0.68), as well as within each order (K ≥ 0.66), is relatively low. The fact that the phylogenetic signal within each clade is lower than the overall phylogenetic signal (with the exception of Platyrrhini) suggests that, although there are differences in endocranial shape among these clades, within each clade phylogeny has less of an impact.
The derived endocranial shape of the platyrrhines appears to be highly influential in defining the morphospace for Euarchontoglires. Anthropoid brains are characterized by a large neocortex, small olfactory bulbs, and a flexed basicranium8,14,15. Reflective of this distinct morphotype, much of the variation captured in PC1 describes variation in these specific features. The unique anthropoid morphotype seen in the platyrrhines is likely the result of multiple evolutionary forces operating on the brain itself, as well as on the cranium which surrounds it. Strong selection for more complex cognitive and sensory processing led to larger neocortices16,17 which required significant modification to the cranium15. Flexion of the basicranium in primates may have resulted from the challenges of packing a larger brain into the cranium15,18,19. Similarly, the relatively smaller olfactory bulbs are suggested to be the product of reduced reliance on olfaction20 as well as the progressively narrower space for the olfactory bulbs as orbit size increased21 while the face was reoriented to accommodate the larger brain18,22.
Across PC 1, strepsirrhines possess an intermediate endocranial shape occurring between the rest of Euarchontoglires and platyrrhines, though not overlapping with either. The distinction between strepsirrhines and anthropoids has been identified in volumetric analyses of the relative neocortex23,24 and olfactory bulb size14,17,25. Recent analyses of endocranial shape within Primates found a clear separation between haplorhines and strepsirrhines with no distinction between Lorisiformes and Lemuriformes9, as is found here. Although occupying distinct areas of the morphospace (Fig. 1), Scandentia and Dermoptera overlap on various PCs (i.e. PCs 2 and 4) with Strepsirrhini, but are more distinct from Platyrrhini. This pattern shows the distinctiveness of the anthropoid brain compared to its euarchontan kin and highlights the degree to which the strepsirrhines form a bridge between higher primates and the rest of Euarchonta in the form of their brain.
Lagomorphs also appear to be highly derived in endocranial shape, with two apparent morphotypes relating to leporids and ochotonids with differences nearing significance. The only lagomorph species which occurs within the morphospace of another clade (Rodentia) is the pygmy rabbit (Brachylagus idahoensis), which possesses a cranial morphotype distinct from the rest of Leporidae26. Not much is known about the adaptive significance of brain morphology in Lagomorpha, but it is possible that this distinct endocranial shape is the byproduct of changes in the cranium rather than selection on brain morphology itself. For instance, lagomorphs have a larger lens (and correspondingly larger eyes and orbits) relative to body weight compared to other mammals27. The constricted circular fissure, which characterizes endocranial shape for this group, may be the result of packing larger eyes into the cranium. An extreme case of this may be seen in the tarsiers in which the cribriform plate and the termination of the olfactory bulbs exists at the end of a long narrow stalk due to the immense size of the orbits. In regard to the separation between leporids and ochotonids, the former is characterized by significant cranial kinesis, thought to be an adaptation to cursoriality28, which may contribute to the morphological distinction between the two families.
Rodents show the greatest dispersion across the morphospace and overlap with all other groups across PC1 (except for primates), possessing a comparatively small neocortex, large olfactory bulbs, and an unflexed basicranium. Across other dimensions (i.e., PCs 2, 3, 4), rodents overlap with all groups. Correspondingly, identification of a unique rodent morphotype is more difficult than in the case of platyrrhines, strepsirrhines, and lagomorphs. This variation may relate to the speciosity and ecological diversity of this order. Several analyses have found correlations between brain regions, including the neocortex, olfactory bulbs, and petrosal lobules, and locomotor behavior in rodents29,30,31,32,33,34. Similarly, analysis of cranial metrics and shape also found strong correlations with locomotor behavior35,36,37. The strong correlation that exists between the shape of the neurocranium and both the basicranium and face make identification of the main source variation (i.e. selective pressure on the brain versus the cranium) difficult to determine, but are worth continued investigation.
The results presented here indicate that allometry has a small but significant impact on endocranial/brain shape across Euarchontoglires. As predicted, shape changes differently with size among these clades (Scandentia, Platyrrhini, Strepsirrhini, Rodentia, and Lagomorpha) and to different extents. This is documented by the distinct R-squared for each clade within the linear pANOVA model, the different slopes, vector lengths, and angles identified in the HOS test, and can be visualized in the regressions of PC1 against size (Fig. 2b), as well as the distinct changes in morphology associated with changes in size within each clade (Fig. 2C–G). This suggests that brain shape allometry is highly flexible within this superorder, and that this diversity in endocranial shape is reflective of evolutionary forces outside of allometric influence. The distinct allometric scaling patterns seen here complement volumetric research which has found that the relationship between brain size and body size is highly variable across Mammalia6,7. The results here also highlight the importance of investigating group specific allometries prior to using common allometric corrections, as even closely related groups may have different allometric patterns (e.g., Platyrrhini and Strepsirrhini).
Though the allometric impact remains significant across the entire sample in both the phylogenetic and non-phylogenetic models (Table 1), within all clades (except for Rodentia) the impact of size on shape is no longer significant when phylogeny is controlled. As Rodentia has the greatest number of taxa the significant result may reflect higher statistical power. However, this is something that is hard to account for, as some of these clades are more diverse and speciose than others. Additionally, the lack of significance in the phylogenetic model can occur when distinct shape/size trends follow phylogenetic patterns. For instance, lagomorphs have the strongest allometric signal across all clades in the linear model (R2 = 0.41). The high R-squared results from the distribution of size and shape variation between the two families as ochotonids are considerably smaller than the leporids and morphologically distinct (captured by the high phylogenetic signal in size; Table 1). When phylogeny is controlled for, the R-squared value substantially decreases.
Alternatively, when variation resulting from natural selection occurs within lineages, phylogenetic corrections may inadvertently control for that variation, obscuring potentially informative adaptive patterns. For instance, the allometric trend within platyrrhines indicates that increased endocast size is associated with an expansion of the neocortex and a reduction in the relative size of the olfactory bulbs. In light of the possible selective forces operating on these brain regions, it is unlikely that this pattern is simply a by-product of allometric constraints, as might be interpreted from the relatively high R-squared in the linear model (Table 1). Instead, the process of adaptation may be taking advantage of this existing allometric pattern (i.e., following a line of least evolutionary resistance;38). To this effect, previous quantitative genetic analyses of cranial diversification in this group indicate significant selection on cranial morphology39, which may have facilitated the changes in brain shape documented in this analysis. However, when phylogeny is corrected for, the relationship between shape and size is no longer significant for this clade likely owing to the strong phylogenetic signal in size (Table 1). Parsing out the “true” relationship in these scenarios can be difficult, especially for clades like the platyrrhines which display a highly derived morphotype with evidence that morphology is adaptively significant. In these cases, phylogenetic corrections should not be applied without considering how the data may be affected, particularly when phylogenetic patterns are the topic of interest. The results of these analyses demonstrate the complex interplay of natural selection and evolutionary constraint, which can sometimes be obscured in analyses that are performed under certain simple assumptions.
Conclusion
This study of endocranial shape variation highlights the unique anthropoid Bauplan, as documented in previous analyses of shape24 and volume14,17,24,25, placing this variation within a broader phylogenetic context. Though Primates are distinct from other Euarchontoglires in endocranial shapespace, the fact that the strepsirrhines are located between the platyrrhines and other Euarchontoglires suggests that their endocranial morphology may be more in-line with the ancestral condition than previously thought. Unlike other orders, defining a clear morphotype for rodents is more difficult as they overlap with several other clades and are spread across multiple dimensions of shapespace, a pattern of variation which is likely driven by ecological factors modifying an ancestral condition32. However, both of these hypotheses require further testing with fossil material.
These groups are also characterized by distinct patterns of variation with size (i.e., allometric trajectories). The existence of these distinct shape trajectories supports Tsuboi et al.’s6 argument that variation within Mammalia is facilitated by reduced allometric constraints allowing for shifts in the trajectories. In some groups allometric constraints may remain weak (i.e., Rodentia) while in others (i.e., Platyrrhini), once a trajectory is established, they may constitute lines of least evolutionary resistance facilitating rapid adaption38. Ultimately, these analyses highlight the value of assessing morphological variation in relation to allometric patterns, as different groups possess distinct trajectories, reflective of their unique evolutionary histories.
Materials and methods: data collection
Endocasts of 140 species of extant Euarchontoglires (Platyrrhini [n = 16], Tarsioidea [n = 2], Strepsirrhini [n = 20], Scandentia [n = 14], Dermoptera [n = 2], Rodentia [n = 71], Lagomorpha [n = 15]) were landmarked with 28 fixed landmarks and two curves of semi-landmarks along the mid-sagittal aspect of the neocortex and cerebellum. Endocasts were produced in previously published projects12,29,30,31,32,33,40 and are available on Morphosource. The landmarks used for this analysis were developed to capture variation among six major brain regions visible on the surface of the endocast (the neocortex, paleocortex, olfactory bulbs, cerebellum [and petrosal lobules], and brainstem) in a morphologically diverse group of Euarchontoglires (33; Table S10, Figs. S4 and S5). This landmark set was found to be highly reproducible, with low levels of intra- and inter-observer error (see33). The anthropoid sample is comprised exclusively of platyrrhines as sexual dimorphism of endocranial shape for catarrhines has not been sufficiently examined and unlike catarhines, platyrrhines exhibit little sexual dimorphism41.
Materials and methods: statistical analyses
Procrustes transformations were applied to the raw coordinate data and a Principal Component Analysis (PCA) was used to visualize variation in endocranial shape among these clades. Surface warps of the species closest to the mean shape (Paraxerus cepapi; USNM 367956) representing shape deformations at the positive and negative extremes of the principal component axes were generated to show shape changes across the morphospace. A broken stick model was used to determine the cut-off for the number of principal components (PCs) to visualize in the PCA42. Given the distinct morphologies of the platyrrhines and strepsirrhines33, Primates were separated into these two clades for analysis. Results for the Order as a whole are presented in supplementary information (Table S2). Phylogenetic signal in endocranial shape and size (log centroid size) was assessed using the K-mult under 9999 random residual permutations procedures (RRPP). A K value less than 1 suggests that endocranial shape between relatives is less similar than expected under a Brownian motion43. A Procrustes ANOVA was applied to these data to assess the relationship of clade and endocranial shape, with subsequent pairwise tests to determine which clades differed significantly from each other. Here and in the ANCOVA tests (below), 10,000 iterations of residuals randomization were used for significance testing. Dermoptera and Tarsioidea were removed for this test as there are only two species each within these clades.
To assess the impact of allometry on endocranial shape, phylogenetic and non-phylogenetic Procrustes ANCOVAs were run on the transformed coordinate data (function: procD.pgls). The phylogenetic ANCOVA (pANCOVA) was performed using phylogenetic generalized least squares regressions using a Brownian motion model of evolutionary progression. Within both ANCOVA models, log centroid size was used as the independent variable and clade was included as a covariate (i.e., landmarks ~ size*clade). The phylogenetic tree used for the pANCOVA was obtained from vertlife.org based on 9999 fossil calibrated node-dated trees from which a single tree was obtained using maximum clade credibility44.
A Homogeneity of Slopes (HoS) test was performed to assess the homogeneity (or lack thereof) of allometric slopes. Two competing models were evaluated within the HoS test: a common allometric slope model for all clades, and a unique allometric slope model for each clade. The homogeneity of slopes tests were performed both with and without phylogenetic context. If there is a significant identified interaction between size and clade, suggesting that allometric slopes differ between clades, pairwise tests are applied to assess which clades significantly differed in means, mean vector lengths, and mean angles in degrees. All ANOVA and ANCOVA models were run with 9999 RRPP.
Two methods were used to visualize allometric scaling patterns among these clades. First, the fitted values for the first principal component generated from the unique allometric slope model were plotted against log centroid size to visualize differences among the allometric slopes for these clades. Second, standardized shape scores (regression scores), generated from the common allometry model, were plotted against log centroid size to compare differences in shape for taxa of the same size among these clades. Wireframes representing shape deformations associated with changes in size were generated using the second allometric plotting method (above) for each clade based on separate clade specific Procrustes transformations and shape size regressions. The relationship between size and shape was also assessed using ANOVAs of clade specific Procrustes transformations and log centroid sizes with and without phylogenetic context.
Data availability
All endocasts used for this analysis are available on Morphosource in the following project: https://www.morphosource.org/projects/000424317?locale=en. Landmark data and the R code used to perform these analyses is accessible from the follow link: https://datadryad.org/stash/share/ppHCC6sYc5s9jRzu_CWO5w_VpbpVdrUfbEINq3GAmFI.
References
Pélabon, C. et al. Evolution of morphological allometry. Ann. N. Y. Acad. Sci. 1320, 58–75 (2014).
Hallgrímsson, B. et al. Integration and the developmental genetics of allometry. Integr. Comp. Biol. 59, 1369–1381 (2019).
Zelditch, M. L. & Swiderski, D. L. The predictable complexity of evolutionary allometry. Evol. Biol. 50, 56–77 (2023).
Pélabon, C. et al. On the relationship between ontogenetic and static allometry. Am. Nat. 181, 195–212 (2013).
Burger, J. R., George, J., Ashaki, M., Leadbetter, C. & Shaikh, F. The allometry of brain size in mammals. J. Mammal. 100, 276–283 (2019).
Tsuboi, M. et al. Breakdown of brain–body allometry and the encephalization of birds and mammals. Nat. Ecol. Evol. 2, 1492–1500 (2018).
Smaers, J. B. et al. The evolution of mammalian brain size. Sci. Adv. 7, eabe 2101 (2021).
Jersion, H. J. Digitized fossil brains: Neocorticalization. Biolinguistics 6, 383–392 (2012).
Sansalone, G. et al. Variation in the strength of allometry drives rates of evolution in primate brain shape. Proc. R. Soc. B: Biol. Sci. 287, 20200807 (2020).
Bienvenu, T. et al. Assessing endocranial variations in great apes and humans using 3d data from virtual endocasts. Am. J. Phys. Anthropol. 145, 231–246 (2011).
Bruner, E. Geometric morphometrics and paleoneurology: Brain shape evolution in the genus Homo. J. Hum. Evol. 47, 279–303 (2004).
López-Torres, S., Bertrand, O. C., Lang, M. M., Silcox, M. T. & Fostowicz-Frelik, Ł. Cranial endocast of the stem lagomorph Megalagus and brain structure of basal Euarchontoglires. Proc. R. Soc. B 287, 20200665 (2020).
Lang, M. M., Allemand, R., López-Aguirre, C., San Martin Flores, G. & Silcox, M. T. Approaches to studying endocranial morphology in Euarchontoglires: Assessing sources of error for a novel and biologically informative set of landmarks. J. Mamm. Evol. 30, 1–18 (2023).
Heritage, S. Modeling olfactory bulb evolution through primate phylogeny. PLoS ONE 9, e113904 (2014).
Ross, C. F. & Ravosa, M. J. Basicranial flexion, relative brain size, and facial kyphosis in nonhuman primates. Am. J. Phys. Anthropol. 91, 305–324 (1993).
DeCasien, A. R., Williams, S. A. & Higham, J. P. Primate brain size is predicted by diet but not sociality. Nat. Ecol. Evol. 1, 0112 (2017).
DeCasien, A. R. & Higham, J. P. Primate mosaic brain evolution reflects selection on sensory and cognitive specialization. Nat. Ecol. Evol. 3, 1483–1493 (2019).
Lieberman, D. E., Ross, C. F. & Ravosa, M. J. The primate cranial base: Ontogeny, function, and integration. Am. J. Phys. Anthropol. 113, 117–169 (2000).
Zollikofer, C. P., Bienvenu, T. & Ponce de Leon, M. S. Effects of cranial integration on hominid endocranial shape. J. Anatomy 230, 85–105 (2017).
Williams, B. A., Kay, R. F. & Kirk, E. C. New perspectives on anthropoid origins. Proc. Natl. Acad. Sci. 107, 4797–4804 (2010).
Cartmill, M. The orbits of arboreal mammals: a reassessment of the arboreal theory of primate evolution. PhD thesis. The University of Chicago (1970).
Smith, T. & Rossie, J. Primate Olfaction: Anatomy and Evolution. In Olfaction and the Brain 135–166 (Cambridge University Press, 2006).
Barton, R. A. & Harvey, P. H. Mosaic evolution of brain structure in mammals. Nature 405, 1055–1058 (2000).
Smaers, J. B. & Vanier, D. R. Brain size expansion in primates and humans is explained by a selective modular expansion of the cortico-cerebellar system. Cortex 118, 292–305 (2019).
Harrington, A. R., Silcox, M. T., Yapuncich, G. S., Boyer, D. M. & Bloch, J. I. First virtual endocasts of adapiform primates. J. Hum. Evol. 99, 52–78 (2016).
Kraatz, B. & Sherratt, E. Evolutionary morphology of the rabbit skull. PeerJ 4, e2453 (2016).
Augusteyn, R. C. Growth of the eye lens: II. Allometric studies. Mol. Vis. 20, 427 (2014).
Bramble, D. M. Cranial specialization and locomotor habit in the Lagomorpha. Am. Zool. 29, 303–317 (1989).
Bertrand, O. C., Amador-Mughal, F. & Silcox, M. T. Virtual endocast of the early Oligocene Cedromus wilsoni (Cedromurinae) and brain evolution in squirrels. J. Anat. 230, 128–151 (2017).
Bertrand, O. C., Amador-Mughal, F., Lang, M. M. & Silcox, M. T. Virtual endocasts of fossil Sciuroidea: Brain size reduction in the evolution of fossoriality. Palaeontology 61, 919–948 (2018).
Bertrand, O. C., Amador-Mughal, F., Lang, M. M. & Silcox, M. T. New virtual endocasts of Eocene Ischyromyidae and their relevance in evaluating neurological changes occurring through time in Rodentia. J. Mamm. Evol. 26, 345–371 (2019).
Bertrand, O. C., Püschel, H. P., Schwab, J. A., Silcox, M. T. & Brusatte, S. L. The impact of locomotion on the brain evolution of squirrels and close relatives. Commun. Biol. 4, 460 (2021).
Lang, M. M. et al. Scaling patterns of cerebellar petrosal lobules in Euarchontoglires: Impacts of ecology and phylogeny. Anat. Rec. 305, 3472–3503 (2022).
Fernández Villoldo, J. A., Verzi, D. H., Lopes, R. T., Dos Reis, S. F. & Perez, S. I. Brain size and shape diversification in a highly diverse South American clade of rodents (Echimyidae): A geometric morphometric and comparative phylogenetic approach. Biol. J. Linnean Soc. 140, 277–295 (2023).
Bertrand, O. C., Schillaci, M. A. & Silcox, M. T. Cranial dimensions as estimators of body mass and locomotor habits in extant and fossil rodents. J. Vertebrate Paleontol. 36, e1014905 (2016).
Verde Arregoitia, L. D., Fisher, D. O. & Schweizer, M. Morphology captures diet and locomotor types in rodents. R. Soc. Open Sci. 4, 160957 (2017).
Camargo, N., Machado, L., Mendonça, A. & Vieira, E. Cranial shape predicts arboreal activity of sigmodontinae rodents. J. Zool. 308, 128–138 (2019).
Marroig, G. & Cheverud, J. M. Size as a line of least evolutionary resistance: Diet and adaptive morphological radiation in New World monkeys. Evolution 59, 1128–1142 (2005).
Marroig, G. & Cheverud, J. M. Did natural selection or genetic drift produce the cranial diversification of neotropical monkeys?. Am. Nat. 163, 417–428 (2004).
San Martin-Flores, G., Nagendran, L., Bertrand, O. & Silcox, M.T. Geometric morphometrics on treeshrew cranial endocasts: A comparative analysis of scandentian and plesiadapiforms brain shapes. Society of Vertebrate Paleontology 2018 Program Abstr. 209 (2018).
Aristide, L. et al. Encephalization and diversification of the cranial base in platyrrhine primates. J. Hum. Evol. 81, 29–40 (2015).
Wilson, L. A. et al. Patterns of ontogenetic evolution across extant marsupials reflect different allometric pathways to ecomorphological diversity. Nat. Commun. 14, 2689 (2023).
Blomberg, S. P., Garland, T. Jr. & Ives, A. R. Testing for phylogenetic signal in comparative data: Behavioral traits are more labile. Evolution 57, 717–745 (2003).
Schliep, K. P. phangorn: Phylogenetic analysis in R. Bioinformatics 27, 592–593 (2011).
Acknowledgements
The authors would like to thank Dr. T.B. Viola for his review and comments on the manuscript. We would like to acknowledge Dr. P. Cox, Dr. A. Harrington, Dr. R. Asher, Dr. M. Lowe, and Gabriela San Martin-Flores for access to CT scans and endocasts through the Morphosource database.
Funding
Funding was provided by Ontario Graduate Scholarship to MML, NSERC Discovery Grant to MTS.
Author information
Authors and Affiliations
Contributions
M.M.L., M.T.S. and L.S. contributed to the study conception and design. Data collection was performed by M.M.L., and analysis was performed by M.M.L., C.L.A., and L.S. The first draft of the manuscript was written by M.M.L. and all authors commented on previous versions of the manuscript. All authors read and approved the final manuscript. Project supervised by M.T.S.
Corresponding author
Ethics declarations
Competing interests
The authors declare no competing interests.
Additional information
Publisher's note
Springer Nature remains neutral with regard to jurisdictional claims in published maps and institutional affiliations.
Supplementary Information
Rights and permissions
Open Access This article is licensed under a Creative Commons Attribution-NonCommercial-NoDerivatives 4.0 International License, which permits any non-commercial use, sharing, distribution and reproduction in any medium or format, as long as you give appropriate credit to the original author(s) and the source, provide a link to the Creative Commons licence, and indicate if you modified the licensed material. You do not have permission under this licence to share adapted material derived from this article or parts of it. The images or other third party material in this article are included in the article’s Creative Commons licence, unless indicated otherwise in a credit line to the material. If material is not included in the article’s Creative Commons licence and your intended use is not permitted by statutory regulation or exceeds the permitted use, you will need to obtain permission directly from the copyright holder. To view a copy of this licence, visit http://creativecommons.org/licenses/by-nc-nd/4.0/.
About this article
Cite this article
Lang, M.M., López-Aguirre, C., Schroeder, L. et al. Endocranial shape variation and allometry in Euarchontoglires. Sci Rep 14, 17901 (2024). https://doi.org/10.1038/s41598-024-68390-y
Received:
Accepted:
Published:
DOI: https://doi.org/10.1038/s41598-024-68390-y
- Springer Nature Limited