Abstract
The glass with various concentrations of trivalent rare earth Dy3+ in borate and boro-phosphate host structures, along with alkali and transition metals, was synthesized by the melt-quenching technique. An FT-IR and FT-Raman spectrum reveal the structural features by tracing out the various molecular vibrations of borate and phosphate units. UV–Vis-NIR spectral analysis was used to investigate the effect of RE concentration on linear (Eg, ∆E, n1, and χ(1)) and non-linear (χ(3), n2) optical characteristics. The metal–oxygen bond character is explored in relation to the impact of alkali cations on the χ(3) of alkali borate and boro-phosphate host glasses. Photoluminescence emission spectra obtained in the optical zone show two intense band emissions traced at 482 and 574 nm, which correspond to 4F9/2 → 6H15/2 (electric-dipole transition) and 4F9/2 → 6H13/2 (magnetic-dipole transition), respectively, and one weak emission at 674 nm, which corresponds to 4F9/2 → 6H11/2. The fluorescence decay time was measured and fitted with the characteristics of a non-exponential decay profile. The estimated color coordinates are in the white region of the CIE 1931 chromaticity curve and are quite close to the conventional white illuminant (0.330, 0.330). In addition, the computed yellow-to-blue (Y/B) ratio and correlated color temperature of the prepared photonic glasses could allow tweaking white light emission via varying concentrations and host environments, which might be advantageous for various optical-based device fabrications.
Graphical Abstract

Similar content being viewed by others
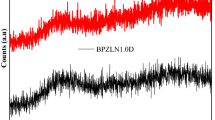
Avoid common mistakes on your manuscript.
1 Introduction
To develop laser science and technology, non-linear optical (NLO) active compounds can advantageously widen the few coherent spectrum sources currently available to far larger spectral regions. Despite its exceptional expandability, there is a strong need to investigate innovative NLO compounds with superior efficiency and performance for prospective photonic applications. The phosphate glasses were primarily studied as hosts for laser and optical amplifiers owing to their high gain properties in comparison to other glass families [1]. The structure of borate glasses is especially fascinating due to the boron anomaly, and as a result, the impact of the network modifier and generated non-bridging oxygens on the linear and non-linear optical properties may be segregated. Because of their considerable effect on luminous efficiency, B2O3-based glasses are widely recognized for their photo-generated second-order NLO effects.
Borate glasses can generate superstructures of B2O3 due to the triangular planer BO3, and tetrahedral BO4 may interact to form diborate (B2O54–), tetraborate/pyroborate (B4O72–), pentaborate (B5O8), or boroxol ring, depending on the quantity of non-bridging oxygens (NBOs) or bridging oxygens (BOs). Alkali metals (Li, Na, and K) modify borate glass, and the emergence of different structural units causes a notable alteration in its physical characteristics [2]. Specifically, lithium borate glasses, which are primarily known as solid electrolytes, are the most adaptable glass systems. One can investigate and synthesize novel materials for optical purposes by adding a specified amount of rare earth oxide to these glasses for specific applications. Host materials with low maximal phonon energies are preferred to synthesize rare earth ions-doped glasses since rare earth ions consume less excitation energy during non-radiative transitions, which leads to relatively high luminous quantum efficiencies. Typically, the maximal phonon energies of the hosts with oxides, such as boron oxides (B2O3) and phosphorous pentoxide (P2O5), are higher than those of the hosts with halide and sulfur. However, the study found that the inclusion of alkali and alkaline metal ions in the oxide glasses substantially reduces phonon energy through energy transfer interactions.
Heavy metal oxide-based RE-infused glass networks have large lasing abilities due to their lower field strength and higher mass and polarizability [3]. The doping concentration of Dy3+ should be considered because it affects both the optical transition properties of the material and the concentration quenching of fluorescence intensity caused by the cross-relaxation between Dy3+ existents. Several researchers reported that concentration quenching occurs at high doping concentrations (≥ 1.0 mol%) of Dy2O3 in glasses, so many have studied the glass systems with only 1.0 mol% of Dy2O3 [4,5,6]. In their ground state, lanthanide ions (Dy3+) have an electronic structure of [Xe] 4f10 5S2 5p6, where the f-electrons are typically non-bonding since they are protected by the 5S2 5p6 orbitals. The optical characteristics are known to be primarily influenced by the configuration and number of 4f electrons. The NLO properties for lanthanide glasses are predicted to be improved because of the optical resonance phenomenon, which is followed by f–f electron transitions. According to reports, the inclusion of TiO2 in glass compositions improves the NLO factors of the glasses due to their empty d-shells and lone pair electrons [7]. The addition of Al2O3 in a sample improves the optical sensitivity by changing the host structure and generating asymmetric sites that result in a 30% increase in the PL intensity when compared to non-Al2O3 samples [8].
The current paper deals with a study of the Dy3+ ion in borate and boro-phosphate host matrixes, together with heavy metal ions. The effects of the Dy3+ doping concentration on the transition, quantum effectiveness, quenching state, and luminescence features were studied. Finally, the values of the CIE chromaticity coordinates are assessed to confirm the w-LED application.
2 Experimental technique
Glasses with the chemical compositions of (60 − x) B2O3 + 25SrCO3 + 7TiO2 + 5LiCO3 + 3Al2O3 + xDy2O3 (x = 0.10, 0.25, 0.50, and 1.00 mol%) and 40B2O3 + 20SrCO3 + 20H6NO4P + 7ZnO + 5LiCO3 + (5 − x) P2O5 + 3Al2O3 + xDy2O3 (x = 0.25 and 0.50 mol%), respectively, were synthesized by melt quenching technique. The boron oxide (B2O3) and phosphorous pentoxide (P2O5) act as a network former in the respective glasses; SrCO3 acts as a network modifier; aluminum oxide (Al2O3) will increase network connectivity; ZnO will increase the mechanical stability of the glass. The measured quantity of AR-grade chemicals was taken and mixed thoroughly to attain homogeneity in an agate mortar. The blended chemicals were placed in an alumina crucible and heated in an electric furnace for 7 h at 1125 °C. After quickly pouring the melt onto a preheated brass metal plate, the samples were annealed at 400 °C to reduce internal stress and achieve structural stability. For convenience, the borate host glasses are designated as BDy0.10, BDy0.25, BDy0.50, and BDy1.0, and the boro-phosphate host glasses are designated as BPDy0.25-and BPDy0.50-based on the Dy3+ concentration.
3 Characterization techniques
3.1 FT-IR spectral analysis
Thin pellets of approximately 3 mm in thickness were made by mixing KBr, and powdered glass samples were subjected to FT-IR measurements. The spectra were taken at room temperature with a JASCO FTIR 6100 spectrometer with a resolution of 0.5 cm−1 in the 400–4000 cm−1 wavenumber range. The FTIR spectra of borate glass systems are depicted in Fig. 1a. The peak at 702 cm−1 confirmed the bending vibration of the B-O-B linkage of the penta-borate unit and the vibration of linking oxygen atoms (BOØ2−). The presence of B-O bond stretching vibrations of tetrahedral BO4 units is responsible for the small band at 870 cm−1 [9]. The peak at 1020 cm−1 is caused by pentaborate as well as BO4 tetrahedral vibrations [10]. The peaks at 1214 cm−1 and 1346 cm−1 correspond to B–O asymmetric stretching vibrations in ortho-borate groups and B–O stretching vibrations of BO3 trigonal units in ortho-, pyro-, and meta-borate units, respectively. A change in the oxygen-to-boron ratio is enhanced by the substitution of B2O3 with a significant concentration of metal oxides such as TiO3 and Al2O3, along with an increase in Dy2O3 concentrations, resulting in stable BO4 units. The creation of BO4 units results in tightly packed glass structures, and it decreases the absorption intensity [11]. It was determined that there were no –OH molecules present in the BDy0.10, BDy0.25, and BDy1.0 glasses because this IR band of these materials did not explore the peak associated with the OH vibration position. In BDy0.5 glass, there are two distinct small-intense peaks at 2852 and 2928 cm−1 showing the presence of a smaller quantity of OH molecules. The weakened OH content indicates improved quantum efficiency and lower non-radiative losses for the RE-doped luminous materials [12]. The presence of water molecules or hydroxyl-related bands is further validated by the significant peak that occurred at 1740 cm−1 in BDy0.5 glass.
Figure 1b demonstrates the vibrational spectrum of BPDy0.25 and BPDy0.50 glass systems. The peak at 692 cm−1 is instigated by P–O–P bending vibrations, and the band at 1038 cm−1 is formed by the symmetric stretching vibrations of the B–O–P bridge between borate (BO4) and phosphate (PO4) units, as well as the B–O stretching in BO4 tetrahedra [13]. The well-defined small band at 1386 cm−1 dominates the infrared spectrum of boro-phosphate glass matrix in the 800 to 1600 cm−1 spectral range as the predominant contribution of Q2 ([P(OP)2(O−)2]) and BO3 units. The minor peaks traced between 2000 and 2500 cm−1 confirm the presence of a hydroxyl group.
3.2 Raman spectral analysis
The FT-Raman spectra were taken with a Bruker RFS 27 Multi-RAM spectrometer with a resolution of 2 cm−1. Because of their intriguing properties, such as the boron anomalies, B2O3-based glasses have received a lot of attention over the years. The Raman spectrum of BDy0.5 glass reveals a significant number of bands caused by the vibration of BO4 and BO3 groups, as well as complexes including [BO3]3– and [BO4]5– groups. The spectrum, as shown in Fig. 2a, reveals a significant intensity band at 102 cm−1, which is associated with the symmetric stretching vibration of heavy metal ions, such as Sr–O and Ti–O bonds. The small-broaden peak at 459 cm−1 is ascribed to B–O–B ring angle bending, which is seen at 470 cm−1 for pure B2O3. The asymmetric structure of a highly intense peak centered at ~ 852 cm−1 is caused by B–O–B symmetric stretching in pyro-borate units [14]. Three peaks were detected through band deconvolution at 709, 794, and 870 cm−1, respectively. The modest intensity band at 709 cm−1 is accredited to the γ-vibration of [BO3]3–/symmetric breathing vibration of boroxol rings with two BO4 groups. The presence of a six-membered boron (boroxol) ring [15] in the prepared glass is indicated by the Raman band nearly observed at 800 cm−1 (in our case 794 cm−1). The 794 and 870 cm−1 peaks were due to symmetric breathing vibrations of the boroxol ring containing one BOx unit (penta-, tetra-, or tri borate), and the symmetric stretching of [BO3]3– units, respectively [16, 17]. Peaks at 993 and 1281 cm−1 indicate that the borate host glass contains B–O– symmetric stretching vibrations in penta-borate (B5O8–) and pyro-borate (B3O54–) anionic groups, respectively [14, 18].
The first band edge in the region of 1200–1450 cm−1, specifically at 1208 cm−1, is accountable for the asymmetric stretching vibration of [BO3]3– units. The band shoulder at 1353 cm−1 is associated with the BØ2O– triangles linked to BØ4– units (O–: non-bridging oxygen atom, and Ø: bridging oxygen atom between the two boron atoms) [19]. The peak at 1421 cm−1 is due to the B–O stretching vibrations of the meta-borate (BO2–) chains and rings. The bands in the Raman spectra of BDy0.5 glass may have widened due to the anharmonicity of the interaction between the BO4 groups in the borate rings and the Li-ions. A strong network structure is created because the emergence of BO4 units in the case of lithium ions is greatly influenced by the mass of the alkali ion [20]. The emergence of a peak after 1900 cm−1 may be due to the presence of hydroxyl group vibration in the glass system.
The Raman spectrum of BPDy0.5 is depicted in Fig. 2b, and when P2O5 is replaced with B2O3, the structural network of this glass exhibits consequently significant modifications. A significant intense peak detected in the lower wavenumber region at ~ 91 cm−1 reveals the vibration of heavy metal ions with the binding oxygen, and it was observed nearly identical location in BDy0.5 glass. The weak vibrations brought on by PO2 bending and bending motions in O–P–O linkages are discernible at 238 and 318 cm−1, respectively [21]. The broad band with a sharp edge at 463 cm−1 and the consecutive band at 574 cm−1 were attributed to the independent di-borate group vibrations, and O–P–O bending vibrations of PO43– tetrahedral units [22, 23]. The band at 713 cm−1 originates from the P–O–P symmetric stretching vibrations, which are produced by the binding oxygen atoms connecting the neighboring PO4 tetrahedral units. The strong and intense peak at 1018 cm−1 is responsible for the P–O symmetric stretching vibration of the Q1 terminal group. The observed band with a center at 1018 cm−1 is asymmetric, and the bulge in the higher wavenumber zone is caused by the overlap of the ~ 1130 cm−1 and ~ 1230 cm−1 bands, which are the locations of the symmetric and asymmetric stretching of non-binding (PO2) of Q2 units, respectively [24]. The deconvoluted peak at 995 cm−1 within a large band is designated to the orthophosphate (PO43–) group symmetric stretching vibration of the Q0 units [23]. The detectable band at 1290 cm−1 may be associated with the combined vibration of boro-phosphate groups [24]. The moderate peak identified at 1338 cm−1 is caused by the P = O stretching vibration of terminal oxygen. The tiny-band edge detected at 1434 cm−1 is revealed for the stretching vibration of molecular oxygen bands and B–O in non-bridging oxygen of [BO3]3– triangles. The peak emergence in the higher region around 1600 cm−1 is ascribed to the OH-bending vibration. Tables 1 and 2 show a description of the vibrational bands found in the FT-IR and FT-Raman spectra of the prepared glasses.
3.3 UV–Vis spectral analysis: optical characterization
Optical absorption spectra of the BDyx (x = 0.10, 0.25, 0.50, and 1.00 mol%) and BPDyx (x = 0.25 and 0.50 mol%) glasses were performed in the JASCO V-670 spectrometer at a wavelength between 190 and 1400 nm at room temperature. Figure 3a and b depicts the collected spectra of the Dy3+-doped borate and boro-phosphate host glasses, respectively. The optical absorption spectra of Dy3+-doped glasses are divided into two sets of manifolds in the UV–Vis (350–550 nm) and near-infrared (715–2000 nm) ranges [25]. The comparatively strong spin-allowed transitions that dominate the near-infrared region of the spectrum are its defining feature. Four absorption bands are originating from the ground state (6H11/2) of the Dy3+ traced out in the intermediate spectral region of both the glass matrixes. The absence of bands in a lower wavelength region (UV–Vis) is caused by the strong absorption of the hosts via the closed packing of heavy metal ions. In addition, it was observed that the absorption peak intensity increased and resolved their positions while increasing the Dy3+ ion concentration in the glass matrices. The four observed dominant absorption band locations and their excited states are as follows: 801 nm (6F5/2), 900 nm (6F7/2), 1086 nm (6F9/2), and 1262 nm (6F11/2). The positions of the absorption bands in the two host glasses are nearly identical. The ligand environment has an impact on the location and intensities of several transitions of Dy3+ ions. The transition 6H11/2 → 6F11/2 has a relatively high intensity compared to the others, and it is termed to be a hypersensitive transition that obeys the selection rules ∆L ≤ 2, ∆ ≤ 2, and ∆S = 0. The visible region’s bands are spin-prohibited and have a weak cross-section, at least up to 400 nm, and thus pose a serious obstacle to the emergence of effective visible laser media.
The optical band gap energies are calculated using the diffused reflectance data of BDyx and BPDyx glasses in the Kubelka–Munk (K-M) theory [26]. The K-M function F(R) in this case is related to the absorption coefficient (α), diffusion coefficient (S), and reflectance (R) of an infinitely thick layer, as given below:
Here, the values of γ = 2 and ½ for indirect and direct transitions are measured by extrapolating the linear sections of the curve to intersect the hυ axis at (F(R)hυ)1/2 = 0 and (F(R)hυ)2 = 0, respectively, as shown in Figs. 4 and 5. The calculated values of direct and indirect band gap energy of the glasses vary from 3.81 to 3.87 eV and 2.73 to 3.33 eV, respectively, with an increase in Dy3+ concentration in borate host matrixes (BDyx), as shown in Figs. 4a and 5a. At initial, the band gap was low as Dy2O3 generated non-bridging oxygens in the borate host matrix; the optical band gap was increased with the further addition of Dy2O3, which was formed by the substitution of boron ions (0.85 Å) with greater atomic radii (0.91 Å) Dy3+ ions. The optical characteristics of the glasses changed when Dy3+ ions were added to the lattice owing to more energy levels being generated within the band gap. In the borate glass matrix, the band gap energy increases with the Dy3+ ion concentration (Table 3) by contributing additional conduction electrons, which then occupy the conduction band at energies greater than the conduction band minimum. As a result, the larger energy differences between conduction and valence bands also influenced the absorption and emission. The energy difference between these bands in an indirect band gap material also includes the change of electron momentum, which is associated with the presence of phonon.
This behavior change could be associated with structural changes in the matrix that modify binding strength. However, as the concentrations of Dy3+ in the boro-phosphate host matrixes increase, the band gap energies decrease from 3.68 to 3.62 eV (direct band gap) and 2.82 to 2.75 eV (indirect band gap), as shown in Figs. 4b and 5b. According to Safeya et al., the rate of conversion of [BO3] → [BO4] increases the inclusion of modifier oxides into the borate host structure [27]. Whereas in the phosphate host, the inclusion of modifier oxides results in the high formation of negative non-bridging oxygen, which is balanced with the positive cations.
In an amorphous structure, the absorption edge indicates the presence of inter-band transition tails, and Urbach energy (∆E) is a crucial factor that determines the level of disordering in the networks; it was calculated using the relation:
The quantity of ∆E signifies the width of localized states, including vacancies, defects due to concentrations, and dangling bonds. As a result, determining the Urbach energy and characterizing the degree of material imperfection are made possible by analyzing the optical absorption spectrum region corresponding to the density of state tails. The Urbach energy measures the steepness of the absorption beginning close to the band edge and, in consequence, the width of the density states. The Urbach energy (∆E) values (~ 0.80 eV) were measured from the slope of the linear section of both the glass matrixes and are displayed in Fig. 6a and b. Table 3 displays the measured Urbach’s energy values of both BDyx and BPDyx glass matrixes. The measured ∆E value decreases in borate host glasses, whereas its value increases in boro-phosphate glasses with an increase in Dy3+ ion concentrations. The low value of ∆E values suggested that the prepared borate host structures have a relatively high organized structure, and it is indicated by a stronger absorption onset. Since h energy and the band gap energy Eg have an inverse relationship, a sample with a larger band gap is anticipated to have a smaller band tail. Furthermore, the lower Urbach energy of the borate-host glasses implies a reduced disorder of phonon states, hence enhancing the emission features including its purity. The increase in ∆E values in BPDyx glasses is due to the increased quantity of non-bridging oxygens and is ascribed to the short-range order of their structure.
The linear refractive index (n) was calculated from the indirect optical band gap energy (Eg) of the glasses using:
The refractive index dispersion of BDyx and BPDyx glasses is shown in Table 3. It is noticeable that increasing the concentration of Dy3+ ions increased the refractive index. According to Wemple, materials with a covalent nature display high microscopic oscillator strength due to the substantial band-broadening of the orbitals of molecules, which results in a high refractive index [28]. Therefore, BDy0.25 and BPDy0.50 in borate and boro-phosphate host matrixes have a high refractive index compared to other prepared glasses in their hosts. Fanderlik [29] reported that the refractive index of the glass is directly related to the glass density, and the glass containing Al2O3 has a comparatively large ion size as well as acting as a glass modifier and not a glass former in the studied glasses. The presence of Al3+, Zn2+, Ti2+, and Sr3+ ions is responsible for the greater levels of density and electronic polarizability shown in these glass systems. For the boro-phosphate (B2O3-P2O5) host glasses with free –OH groups, a higher index of refraction and more non-bridging oxygen generation lead to a denser structure. Many authors discovered that the system containing Li2CO3 exhibits the highest degree of refraction. Because the refractive index is affected not only by polarizability but also by the molar volume M/ρ, and as a result, a dense structure is predicted as the molar volume decreases [30, 31]. The high refractive index of transition metal-containing glasses including Sr2O3 and Ti2O may be due to these ions’ ability to reduce the average oxygen coordination number. This causes the structure to become more compact, which is then reflected in the rise in refractive index.
The two-phonon absorption (TPA) coefficient is an important metric that may be determined by employing indirect optical band gap energy values, and it was used to evaluate the optical switching performance in waveguides [32].
TPA coefficient of composition BDy1.0 glass is low (9.787 cm/GW), implying their application in waveguides, and measured TPA values (presented in Table 3) demonstrate that TPA may be modified by influencing the optical band gap [33].
3.4 Non-linear optical characterization
Non-linear optical features are caused by non-linear electric polarization, which can be described as follows:
where P represents the electric polarization, and χ represents optical susceptibility. In a glass structure with inversion symmetry, χ(1) is theoretically zero. As a result, the non-linear optical response in glass is dominated by χ(3). The addition of RE ions to the glass matrix affords them a non-linear optical characteristic. The following equation relates first-order linear optical susceptibility χ(1) and third-order non-linear optical susceptibilities χ(3) and linear refractive indices (n1) and non-linear refractive indices (n2) [34]:
Figure 5b depicts the variation of χ(3) and n2 with the various concentrations of Dy3+ ions in the BDyx glasses. Miller’s rule states that the greater the refractive index, the greater the value of χ(3) [35]. As a result, the high χ(3) in BDy0.25 from the borate host and BPDy0.50 from the boro-phosphate is caused by the covalent nature of the metal–oxygen bond. The non-linear behavioral patterns of the curves suggested that the concentration of Dy3+ influenced the non-linear optical characteristics of prepared glass systems. Alkali cations can influence the total χ(3) value in alkali borate and boro-phosphate glasses because χ(3) for pure B2O3 glass is quite small (= 2.88 × 10−14 esu) [36], as shown in Table 3. Adir et al. expected that the glasses containing ions with high polarizability would exhibit a substantial χ(3) value. The fact that the value of χ(3) steadily rises as Eg falls suggests that Eg is the key factor of the χ(3) value of all the glasses in the present work. Due to the reduced band gap, there can be an impact caused by optical resonance enhancement. Dysprosium-doped boro-phosphate glasses exhibit higher optical non-linearity than borate glasses due to one-photon absorption [37]. The combined contribution of the Dy3+ ion and heavy-metal oxide ions (Ti2+ and Sr3+) with high polarizability could further increase χ(3).
The two types of resonance effects that rise in χ(3) value are the pre-resonance effect and the rigorous-resonance effect. The rigorous resonance effect termed as χ(3) values is enhanced dramatically due to the large transition moment to the first excited state. According to Nasu et al. [38], the field strength of rare-earth cations has a significant impact on the non-linearity. The large optical non-linearity is caused by the small field strength ions’ inability to bind valence electrons as tightly. The optical band gap value for these glasses is substantially higher than the energy of a photon in the third harmonic beam (E = 1.96 eV). As a result, the pre-resonance effect can be the primary factor in increasing the χ(3) in both the host glasses. Table 3 illustrates the linear and non-linear optical parameters of BDyx (x = 0.10, 0.25, 0.50, and 1.00) and BPDyx (x = 0.25 and 0.50) glasses. The calculated non-linear refractive index (n2) value of BDy0.25. BPDy0.25 and BPDy0.50 glasses were found to be higher than the other prepared glasses which suggests that these glasses can be utilized in light-regulated optical devices.
3.5 Photoluminescence
The excitation spectra of all the samples were monitored for the emission wavelength of 574 nm. The spectra reveal three predominant peaks at 350, 367, and 388 nm and other minor peaks at 327, 340, 427, 454, and 475 nm, which correspond to transitions of Dy3+ ions from the 6H15/2 state to the 4M15/2 + 6P7/2, 4I11/2, 4I13/2 + 4F7/2, 6P3/2, (4F,4D)5/2, 4G11/2, 4I15/2, and 6F9/2 states, respectively, as shown in Fig. 7. The emission spectra were recorded in the 400–700 nm range for the excitation wavelength of 388 nm and are shown in Fig. 8. There are a large number of f-block energy states in Dy3+ with 4F9 meta-stable configuration. The luminescent spectrum reveals two strong emission bands at 482 nm due to magnetic-dipole (MD) transition 4F9/2 → 6H15/2 (blue), and 574 nm corresponds to the electric-dipole (ED) transition 4F9/2 → 6H13/2 (yellow) strongly influenced by the surrounding environment. The emission at 662 nm is attributed to 4F9/2 → 6H11/2 (red) transitions and is found to be relatively weak. It was discovered that the emission intensity of the ED transition is greater than the MD transition for the present glasses, and it follows the selection rules ∆L = ± 2 and ∆j = ± 2. However, in comparison to the other two identified emission bands, the emission band in the 662 nm has a low intensity. Among those observed, the 4F9/2 → 6H13/2 transition is hypersensitive, and the ligand field surrounding the Dy3+ ion site has a significant impact on its strength. Typically, the host has a minute influence on the intensity of the hypersensitive transition, or it changes only slightly from host to host, whereas the intensity of the magnetic-dipole transition varies significantly from host to host [39]. Additionally, the emission of white light has a greater influence on the intensity variation between transitions. The color of the luminescence is nearly white, if the emission intensities of the two transitions (4F9/2 → 6H15/2, 6H13/2) are identical, for instance, in the systems Ba3Bi(PO4)3:Dy3+, BaY2ZnO5:Dy3+, and (Gd0.98Dy0.02)(BO2)3 [40, 41].
In borate glass system, the intensity of luminescence emission is directly related to their indirect band gap energy. The prepared glasses containing 0.5 and 1 mol% of Dy3+ ion concentration have a relatively large band gap. These samples absorb large quantities of high-energy photons, which cause the high-intensity emission. The wider energy gap below the 4G5/2 state of Dy3+ ion-doped glass substantially reduces the multi-phonon transition rate, even in glass with high-phonon energy. Moreover, the elevated energy states of Dy3+ ions impact three emission bands, leading to emission within the visible region.
From Fig. 8, it can be observed that the luminescence intensity rises as the Dy3+ ion concentration rises to 0.5 wt% and 0.25 wt% in borate and boro-phosphate host glasses, respectively. As the concentration of Dy3+ ions increases in the glass network, the inter-ionic distance decreases gradually, and the exchange interaction among the Dy3+ ions becomes robust. When the inter-ionic distance of Dy3+-Dy3+ ions is smaller than the critical distance, the tunneling effect is easily activated, leading to cascade energy transfer and luminescence quenching [42]. Figure 9 illustrates the schematic representation of the concentration quenching mechanism in Dy3+-doped host glasses.
Z. Zhu et al. reported that a multi-polar interaction plays an essential role in the concentration quenching mechanism of Dy3+ [43]. According to Van Uitert’s report, the relationship between activator ion concentration and their emission intensity can be represented as follows [44]:
where x = concentration of activator ion; I = intensity of emission; k and β are constant for a given host network under the identical excitation condition; and θ = 6, 8, and 10 values correspond to the different multi-polar interactions. Considering that β(x)θ/3 > > 1, then Eq. (10) can be rewritten as:
The relation between log (x) and log (I/x) and the accompanying straight line has a slope that signifies − θ/3, as shown in Fig. 10a and b for BDyx and BPDyx glass, respectively. Herein, the computed average value (both the emission) of θ/3 is 0.718, and θ can be measured as 2.155 for BDyx glass, whereas the value is 1.150, and θ can be measured as 3451 for BPDyx glass. The θ value for both glass matrixes was found to be less than 6. The finding indicates that the dipole–dipole (D-D) interaction influences the concentration quenching mechanism of Dy3+ emission in borate and boro-phosphate hosts [43, 45]. Table 4 specifies the magnitude of − θ/3 for the individual emission bands of both host glasses.
3.6 Y/A ratio and decay profile
The dominance of yellow emission is the result of a hypersensitive transition, which specifies that the Dy3+ ions are positioned at low-symmetry sites without an inversion center. The Y/B value is proportional to the Dy3+ concentrations, and it is found to be 1.233–1.352 and 1.218–1.298 for the BDyx (x = 0.10, 0.25, 0.50, and 1.00) and BPDyx (x = 0.25 and 0.50) glasses, respectively. According to Arunkumar et al. [46], a rise in the covalency between Dy3+–O2− ions and the atoms surrounding them in the glasses matrix is indicated by a ratio of Y/B increases. As a result, while the concentration of Dy3+ ions in the prepared glasses increases, it consequently increases the covalency of the Dy–O bond. The value of Y/B changes when Dy3+ is introduced in place of an element with a different valency (Al3+/2+, Li3+, Sr2+, Ti2+, and Zn2+), and this change is proportional to variations in the concentration of Dy3+. The local symmetry of Dy3+ will change with an increase in Dy3+ concentration because defects are produced in this circumstance. According to the gradual increase in Y/B ratios, the present glasses can produce blue or white light depending on the concentration of Dy3+ [47, 48].
The PL decay curves of BDyx (x = 0.25 and 0.50) and BPDyx (x = 0.25 and 0.50) glasses are displayed in Fig. 11. For all the prepared glass networks, the decay rates of emission exhibit a non-exponential configuration. The Dy3+ non-exponential decay curves were fitted to the subsequent tri-exponential function:
where, τ1, τ2, and τ3 are the fluorescence lifetime components that undergo calculating the average lifespan, while A1, A2, and A3 stand for the amplitudes of the corresponding decay components. The expression that follows can be used to determine the average lifespan for a non-exponential with three components [49].
The Dy3+ ion concentration influences the 4F9/2 fluorescence lifetime. For BDyx glasses, the mean lifespan values decreased from 1.490 to 1.190 ms with an increase in Dy3+ dopant concentration; however, the value of 1.082 ms remained the same for BPDyx glasses. Effective lifetimes tend to be shortened as Dy3+ concentration rises; this is likely due to energy transfer within luminescence quenching centers, via the cross-relaxation (CR) process, and energy transfer from impurities [50]. In the present study, this behavior is explained by a substantial quenching as the dopant concentration increases and the distance between consecutive ions decreases. Therefore, there is a higher chance that the resonant interaction of energy within the Dy3+ ions will increase, leading to quicker fluorescence decay [51]. The non-radiative transmission of energy via cross-relaxation is schematically represented in Fig. 12. Three cross-relaxation channels could be discovered based on the energy resonance [2]. The excited Dy3+ ions relaxed from 4F9/2 level to 6F11/2 (CH-1), 6F5/2 (CH-2), or 6F3/2 (CH-3) level by transmitting its energy non-radiatively to another Dy3+ ions in 6H15/2 level (ground state) and the energy acquired Dy3+ ions excite to 6F3/2 (CH-1), 6F9/2 (CH-2), or 6F11/2 (CH-3)state. All the Dy3+ ions will relax to their ground state non-radiatively which results in the luminescence quenching.
The Inokuti-Hirayama model was used to evaluate the decay curve to explicate the energy migration between Dy3+-Dy3+ ions [26].
\({I}_{0}\) is the initial emission intensity; τ represents the intrinsic lifetime of donors, and Q is the energy transfer parameter, which is expressed as follows:
where \(\Gamma\) is Euler’s gamma function, and S is the multipolar interaction parameter. NA represents Dy3+ concentration, and Rc denotes critical transfer distance. Figures 13 and 14 show the Inokuti-Hirayama fit for the decay profile of the BDyx and BPDyx (x = 0.25 and 0.50) glasses. The donor–acceptor ion interaction parameter (CDA) and the shortest distance between the randomly distributed RE ions can be determined from the following equations [52]:
The cross-relaxation between donor and acceptor could be the predominant reason for non-mono exponential behavior in all the samples. Drandom > > CDA suggests the cross-relaxation and energy transfer probably occur among the Dy3+ clusters ions and not out of randomly distributed ions. Table 5 shows the lifetime value, energy transfer, and other related parameters derived from the PL Decay curve of BDyx and BPDyx (x = 0.25 and 0.50) glasses.
3.7 CIE chromaticity coordinates
The color coordinates are one of the crucial components for assessing the effectiveness of the glasses’ color rendering activity.
where X, Y, and Z stand for the three tristimulus values and can be obtained by:
where \(\overline{x }\left(\lambda \right), \overline{y }\left(\lambda \right),\) and \(\overline{z }\left(\lambda \right)\) are color-matching functions, λ is the wavelength of the analogous monochromatic light, and P(λ) is a spectral power distribution. Another significant factor for better understanding sources of light for LED applications is color purity or color saturation.
where (x,y), (xi, yi), and (xd, yd) represent the luminescent chromaticity coordinates, the standard white emission CIE coordinates (0.330, 0.330), and the dominant wavelength coordinates, respectively. The CIE chromaticity coordinates (x, y) of the present glasses with various concentrations of Dy3+ have been measured from the luminescence spectra and are discovered to (0.312, 0.314), (0.310, 0.313), (0.323, 0.335), and (0.328, 0.344) for BDyx (x = 0.10, 0.25, 0.50, and 1.00), whereas the values are (0.293, 0.293) for BPDy0.25 and (0.317, 0.332) for BPDy0.50 glass. The color purity (Pc) of conventional white light is 0%; the Pc of the BDyx glasses was found to vary between 9.5 and 2.2%, whereas Pc of BPDyx glasses varied between 17.5 and 5.5%. Furthermore, the Pc value of the glasses was found to increase as Dy concentrations increased.
Figure 15 depicts the distribution of chromaticity coordinates in the CIE 1931 chromaticity diagram. The chromaticity coordinates move to the yellow-white zone when Dy3+ concentration increases, indicating that warm white light is generated. Lower quantities of Dy3+ in a glass demonstrated that emission occurred in the blue-white region, as validated by their predominated wavelengths (λd) as well as the color purity (Pc). Correlated color temperature (CCT) is an essential technique for illuminant critical features. The point of white light area achieved for BDy0.5 may be also made to correspond with reference white light by adjusting the excitation wavelengths and calcination temperature. The CCT of prepared BDyx and BPDyx glasses was computed using McCamy’s relation from the chromaticity coordinates (x and y): CCT = − 437 n3 + 3601 n2 − 6861 n + 5514.31. Where n = (x − 0.3320)/(y − 0.1858); x and y are the CIE chromaticity coordinates. Natural white light can be classified into three broad zones based on CCT values: cool white (> 5000 K), pure white (3700–5000 K), and warm white (< 3700 K). A CCT value greater than 5000 K suggests cool white light from the glasses. Table 6 shows that the CCT values of borate host glasses range from 5664 to 6769 K, and those of boro-phosphate host glasses vary from 6237 to 8432 K. CCT values of more than 5000 K were found in Dy3+-doped borate and boro-phosphate glass networks; when compared with recent literature, the prepared glasses showed a cool white light emission.
4 Conclusions
Dy3+ ions doped in two different hosts; borate (BDyx) and boro-phosphate (BPDyx) glasses were prepared by the melt-quenching technique. The vibrational analysis of glasses confirmed that an increase in the concentration of Dy3+ ions increases the conversion of BO3 units into BO4 tetrahedral units, which leads to a decrease in the maximum phonon energy of the glass system and an increase in the intensity of luminescence. The BDy0.5 and BDy1.0 glasses exhibited a lower Urbach energy, indicating a lesser phonon state disruption that leads to excellent emission characteristics. The resonance processes in the borate host matrix cause the BDy0.5 glass to react more prominently in non-linear optical characteristics. The PL spectra showed that a dipole–dipole interaction influences the concentration quenching over 0.5 wt% and 0.25 wt% of Dy3+ dopant in a borate and boro-phosphate glass network, respectively. The Inokuti-Hirayama fit also confirms the dominance of dipole–dipole interaction energy transfer between acceptor–donor ions. With increasing Dy3+ concentration, the bluish-white color of the BDyx and BPDyx glasses shifted to yellowish-white with extreme color purity. Among all the prepared glasses, BDy0.5 had a CCT value of 5942 K and color coordinates of (0.323, 0.335), indicating it is a promising cool-white light emitting glass for optical lighting applications.
Data availability
All data generated or analyzed during this study are included in this published article.
References
J. Gangareddy, H. Syed, S. Chakraborty, P. Sen, M. Ghosh, K.K. Dey, K. Bhattacharyya, K. Annapurna, V.R. Soma, A.R. Allu, Tunable, efficient, ultrafast broadband nonlinear optical properties of TiO2-loaded complex phosphate glasses. Mat. Res. Bull. 167, 112141 (2023). https://doi.org/10.1016/j.materresbull.2023.112414
S. Kaur, D. Arora, S. Kumar, G. Singh, S. Mohan, P. Kumar, P. Kriti, D.P. Singh. Kaur, Blue-yellow emission adjustability with aluminium incorporation for cool to warm white light generation in dysprosium doped borate glasses. J Lumin 202, 168–175 (2018). https://doi.org/10.1016/j.jlumin.2018.05.034
G.A. Kumar, A. Martinez, E. Mejia, J.G. Eden, Fluorescence and upconversion spectral studies of Ho3+ in alkali bismuth gallate glasses. J. Alloys and Comp. 365, 117–120 (2004). https://doi.org/10.1016/S0925-8388(03)00650-9
L. Shamshad, G. Rooh, K. Kirdsiri, N. Srisittipokakun, B. Damdee, H.J. Kim, J. Kaewkhao, Photoluminescence and white light generation behavior of lithium gadolinium silicoborate glasses. J. Alloy. Compd. 695, 2347–2355 (2017). https://doi.org/10.1016/j.jallcom.2016.11.105
J. Kaewkhao, N. Wantana, S. Kaewjaeng, S. Kothan, H.J. Kim, Luminescence characteristics of Dy3+ doped Gd2O3-CaO-SiO2-B2O3 scintillating glasses. J. Rare Earth 34(6), 583–589 (2016). https://doi.org/10.1016/S1002-0721(16)60065-0
L.H. Jiang, Y.L. Zhang, C.Y. Li, J.Q. Hao, Q. Su, Thermoluminescence studies of LiSrBO3: RE3+ (RE= Dy, Tb, Tm, and Ce). Appl. Radiat. Isot. 68(1), 196–200 (2010). https://doi.org/10.1016/j.apradiso.2009.10.001
K. Biswas, A.D. Sontakke, K. Annapurna, Effect of TiO2 on thermal, structural and third-order nonlinear optical properties of Ca – La – B – O glass system. J Alloy Compd 489, 493–498 (2010)
Camila DS. Bordon, Evellyn S. Magalhaes, Davinson M. da Silva, Luciana RP. Kassab, Cid B. de Araújo, Influence of Al2O3 on the photoluminescence and optical gain performance of Nd3+ doped germanate and tellurite glasses. Opt Mat 109, 110342 (2020). https://doi.org/10.1016/j.optmat.2020.110342
B. Hari Babu, V.V. Ravi Kanth Kumar, Warm white light generation in γ-irradiated Dy3+, Eu3+ codoped sodium aluminoborate glasses. J Lumin. 169, 16–23 (2016). https://doi.org/10.1016/j.jlumin.2015.08.058
P.P. Pawar, S.R. Munishwar, R.S. Gedam, Intense white light luminescent Dy3+ doped lithium borate glasses for W-LED: A correlation between physical, thermal, structural and optical properties. J. Sol. State. Sci. 64, 41–50 (2017). https://doi.org/10.1016/j.solidstatesciences.2016.12.009
D. Singh, K. Singh, B.S. Bajwa, G.S. Mudahar, D.P. Singh, Manupriya Manupriya, M. Arora, V.K. Dangwal, Optical and structural properties of Li2O–Al2O3–B2O3 glasses before and after γ-irradiation effects. J. Appl. Phys. 104, 10 (2008). https://doi.org/10.1063/1.3003070
S. Kaur, Amit K. Vishwakarma, Aman Prasad NishaDeopa, M. Jayasimhadri, Spectroscopic studies of Dy3+ doped borate glasses for cool white light generation. Mat. Res. Bull. 104, 77–82 (2018). https://doi.org/10.1016/j.materresbull.2018.04.002
F.H. ElBatal, S. Ibrahim, A.M. Abdelghany, Optical and FTIR spectra of NdF3-doped borophosphate glasses and effect of gamma irradiation. J. Mol. Struct. 1030, 107–112 (2012). https://doi.org/10.1016/j.molstruc.2012.02.049
Sd. Zulfiqar Ali Ahamed, C. Madhukar Reddy, B. Deva Prasad Raju, Structural, thermal, and optical investigations of Dy3+ ions doped lead containing lithium fluoroborate glasses for simulation of white light, Opt. Mat. 35, 1385 – 1394 (2013) https://doi.org/10.1016/j.optmat.2013.02.006
Hideharu Ushida, Yasuhiko Iwadate, Takeo Hattori, Shin Nishiyama, Kazuko Fukushima, Yasuhisa Ikeda, Makoto Yamaguchi et al., Network structure of B2O3–PbO and B2O3–PbO–PbBr 2 glasses analyzed by pulsed neutron diffraction and Raman spectroscopy. J. Alloys Compd. 377(1–2), 167–173 (2004). https://doi.org/10.1016/j.jallcom.2003.12.038
S.R. Rejisha, P.S. Anjana, N. Gopakumar, N. Santha, Synthesis and characterization of strontium and barium bismuth borate glass-ceramics. J. Non-Cryst. Sol. 388, 68–74 (2014). https://doi.org/10.1016/j.jnoncrysol.2014.01.037
B.N. Meera, J. Ramakrishna, Raman spectral studies of borate glasses. J. Non-Cryst. Solids 159(1–2), 1–21 (1993). https://doi.org/10.1016/0022-3093(93)91277-A
C.N. Santos, D.D.S. Meneses, P. Echegut, D.R. Neuvile, A.C. Hernandes, A. Lbanez, Appl. Phys. Lett. 94, 15190–151903 (2009)
R.C. Lucacel, V. Carmen Marcus, I. Timar, Ardelean, FT-IR and FT-Raman spectroscopic studies on B2O3-PbO-Ag2O glasses doped with manganese ions. J. Sol. Stat. Sci. 9, 850–854 (2007). https://doi.org/10.1016/j.solidstatesciences.2007.07.006
B. Deva Prasad Raju, C. Madhukarreddy, Structural and optical investigations of Eu3+ ions in lead-containing alkali fluoroborate glasses Opt. Mat. 34, 1251–1260 (2012). https://doi.org/10.1016/j.optmat.2012.01.027
M. Dhavamurthy, P. Vinothkumar, Manoj Mohapatra, Antony Suresh, Priya Murugasen, Effects of Ce3+/Dy3+ and Ce3+/Sm3+ co-doping as a luminescent modifier in alumina-borophosphate glasses for w-LED applications, Spectchim. Act. Part A: Mol. Biomol. Spect. 266, 120448 (2022). https://doi.org/10.1016/j.saa.2021.120448
M. Anastasopoulou, K.C. Vasilopoulos, D. Anagnostopoulos, I. Koutselas, D.K. Papayannis, M.A. Karakassides, Structural and theoretical study of strontium borophosphate glasses using Raman spectroscopy and ab initio molecular orbital method. J. Phys. Chem. B 121, 4610–4619 (2017). https://doi.org/10.1021/acs.jpcb.7b01563
P. Ramakrishna, P. Sitakanta Panda, R.K. Padhi. Vinodkumar, B. Hrudananda Jena, S. Panigrahi, Structural and optical properties of cerium and dysprosium coactivated borophosphate glasses for cool white light application. J Non-Cryst Sol 566, 120883 (2021). https://doi.org/10.1016/j.jnoncrysol.2021.120883
D. Carta, D. Qiu, Paul Guerry, Ifty Ahmed, Ensanya A. Abou, Jonathan C. Neel, Mark E. Knowles, Robert J. Smith, Newport, The effect of composition on the structure of sodium borophosphate glasses. J. Non-Cryst. Sol. 354, 3671–3677 (2008). https://doi.org/10.1016/j.jnoncrysol.2008.04.009
E. Cavalli, Optical spectroscopy of Dy3+ in crystalline hosts: general aspects, personal considerations and some news. J Opt Mat X 1, 100014 (2019). https://doi.org/10.1016/j.omx.2019.100014
M. Priya, M. Dhavamurthy, A. Antony Suresh, M. MonojMohapatra, Luminescence and spectroscopic studies on Eu3+- doped borate and boro-phosphate glasses for solid-state optical devices. J. Opt. Mat. 142, 114007 (2023). https://doi.org/10.1016/j.optmat.2023.114007
S. Ibrahim, Manal Abdel-Baki, Fouad El-Diasty, Zinc borophosphate glasses for infrared-based optical applications. Opt. Eng. 51(9), 093401–093401 (2012). https://doi.org/10.1117/1.OE.51.9.093401
S.H. Wemple, Optical oscillator strengths and excitation energies in solids, liquids, and molecules. J. Chem. Phys. 67(5), 2151–2168 (1977). https://doi.org/10.1063/1.435102
O. Fanderlik, properties of glass (Elsevier Publishing Company, Amesterdam, 1983)
H. Scholze, Glass nature, structure and properties (Springer, Berlin, 1990)
F.M. Ezz El-Din, N.A. El-Alaily, H.A. El-Batal, Chem. 163, 267–275 (1992). https://doi.org/10.1007/BF02034800
J. Dahiya, A. Hooda, Ashish Agarwal, Satish Khasa, Effect of dysprocium and samarium RE ion co-doping on photoluminescence behavior of novel alkali fluride bismuth borate glasses: a white LED materials. Opt. Mat. 134, 113162 (2022). https://doi.org/10.1016/j.optmat.2022.113162
G. Lakshminarayana, A.N. Meza-Rocha, O. Sariano-Romero, E.F. Huerta, U. Caldino, A. Lira, Dong-Eun Lee, Jonghun Yoon, Taejoon Park, Pr3+-doped B2O3-Bi2O3-ZnO-NaF glasses comprising alkali mixed alkali oxides for potential warm white light generation, blue laser, and E-+S-+C-optical bands amplification applications. J. Mater. Res. Technol. 13, 2501–2526 (2021). https://doi.org/10.1016/j.jmrt.2021.06.037
A.M. Ibrahim, A.H. Hammad, A.M. Abdelghany, G.O. Rabie, Mixed alkali effect and samarium ions effectiveness on the structural, optical and non-linear optical properties of borate glass. J. Non-Cryst. Solids 495, 67–74 (2018). https://doi.org/10.1016/j.jnoncrysol.2018.05.015
J.J. Wynne, Optical third-order mixing in GaAs, Ge, Si, and InAs. Phys. Rev. 178, 1295–1303 (1969). https://doi.org/10.1103/PhysRev.178.1295
K. Terashima, Sae-Hoon Kin, Toshinobu Yoko, Nonlinear optical properties of B2O3-based glasses: M2O–B2O3 (M = Li, Na, K, Rb, Cs, and Ag) binary borate glasses. J. Am. Ceram. Soc. 78(6), 1601–1605 (1995). https://doi.org/10.1111/j.1151-2916.1995.tb08857.x
K. Terashima, Suguru Tamura, Sea-Hoon Kim, Toshinobu Yoko, Structure and nonlinear optical properties of lanthanide borate glasses. J. Am. Ceram. Soc. 80(11), 2903–2909 (1997). https://doi.org/10.1111/j.1151-2916.1997.tb03210.x
H. Nasu, J. Mastsuoka, O. Sugimoto, M. Kida, K. Kamiya, Non-resonant type third-order optical nonlinearity of rare earth oxides – containing GeO2 glasses. J. Ceram. Soc. Jpn. 101, 43–47 (1993). https://doi.org/10.2109/jcersj.101.43
D. Gao, Yunci Li, Lihong Cheng, Shengyi Liu, Sai Xu, Xiangping Li, Jinsu Zhang, Xizhen Zhang, Yongze Cao, Yichao Wang, Xin Wang, Yuhang Zhang, Xuezhu Sha, Li Wang, Baojiu Chen, Concentration effects of fluorescence quenching and optical transtition properties of Dy3+ doped NaYF4 phosphor. J. Alloys and Comp. 895, 162616 (2022). https://doi.org/10.1016/j.jallcom.2021.162616
L. Chih-Hao, Lay-Gaik. Teoh, Kuan Ting Liu, Yee-Shin. Chang, Near white light, emission of BaY2ZnO5 doped with Dy3+ ions. J. Alloys and Comp. 517, 9–13 (2012). https://doi.org/10.1016/j.jallcom.2011.11.088
X. Zhang, Fangui Meng, Wnelan Li al, Sun II Kim, Young Moon Yu, Hyo Jin Seo, Investigation of energy transfer and concentration quenching ofDy3+ luminescence in Gd(BO2)3 by means of fluorescence dynamics. J. Alloys and Comp. 578, 72–76 (2013). https://doi.org/10.1016/j.jallcom.2013.05.012
X. Li, P. Li, Z. Wang, S. Liu, Q. Bao, X. Meng, K. Qiu, Y. Li, Z. Li, Z. Yang, Color-tunable luminescence properties of Bi3+ in Ca5(BO3)3F via changing site occupation and energy transfer. Chem. Mater. 29(20), 8792–8803 (2017). https://doi.org/10.1021/acs.chemmater.7b03151
Z. Zhu, C. Tao, Z. Wang, Z. Yang, P. Li, Luminescence and energy transfer of warm white-emitting phosphor Mg2Y2Al2Si2O12 Dy3+, Eu3+ for white LEDs. RSC Ad 11, 32707–32716 (2021). https://doi.org/10.1039/d1ra06215h
L.G. Van Uitert, Characterization of energy transfer interaction between rare earth ions. J. Elect. Chem. Soc. 114, 1048 (1967). https://doi.org/10.1149/1.2424184
K. Saidi, M. Dammak, Crystal structure, optical spectroscopy and energy transfer properties in NaZnPO4:Ce3+, Tb3+ phosphors for UV-based LEDs. RSC Adv. 10(37), 21867–21875 (2021). https://doi.org/10.1039/d0ra04163g
S. Arunkumar, G. Venkataiah, K. Marimuthu, Spectroscopic and energy transfer behaviour of Dy3+ ions in B2O3-Teo-PbO-PbF2-BiO3-CdO glasses for laser and WLED applications, Spectrochem Acta Part A Mol. Biomol. Spectrosc. 16, 1684–1697 (2015). https://doi.org/10.1016/j.saa.2014.10.067
Q. Su, Zhiwu Pei, Lisheng Chi, Hongjie Zhang, Zhongyi Zhang, Feng Zou, The yellow-to-blue intensity ratio (Y/B) of Dy3+ emission. J. Alloys and Comp. 192, 25–27 (1993). https://doi.org/10.1016/0925-8388(93)90174-L
K. Linganna, Ch Srinivasa Rao, C.K. Jayasankar, Optical properties and generation of white light in Dy3+-doped lead phosphate glasses. J. Quant. Spectrosc. Radiat. Transf. 118, 40–48 (2013). https://doi.org/10.1016/j.jqsrt.2012.12.002
B. Grobelna, A. Synak, P. Bojarski, K. Szczodrowski, B. Kuklinski, S. Raut, I. Gryczynski, Synthesis and luminescence of Dy3+ ions in silica xerogels doped with Ln2-xDyx(WO4)3. J. Opt. Mat. 35, 456–461 (2013). https://doi.org/10.1016/j.optmat.2012.09.040
P. Haritha, I. R Martin, C.S. Dwaraka Viswanath, N. Vijaya, K. Venkata Krishnaiah, C. K. Haranath, V. Lavin, V. Venkataramu, J. Opt. Mater. 70, 16 – 24 (2017). https://doi.org/10.1016/j.opmat.2017.05.002
S. Chemingui, M. Ferhi, K. Horchani-Naifer, M. Ferid, Synthesis and luminescence characteristics of Dy3+ doped KLa(PO3)4. J. Lumin. 166, 82–87 (2015). https://doi.org/10.1016/j.jlumin.2015.05.018
M.E. Alvarez-Ramos, Luminescence and study of channels for cross-relaxation dependent on the concentration of Sm3+ under simultaneous UV-IR excitation in tellurite-germanate glasses. J. Alloys Compd. 854, 157076 (2021). https://doi.org/10.1016/j.jallcom.2020.157076
M. Priya, P. Dhavamurthy, A. Antony Suresh, Manoj Mohapatra, Structural, optical, and thermoluminescence characterizations of 1 mol% Dy3+ ion-activated fluro boro-phosphate glass for photonic devices. Spectchim Act Part A: Mol Biomol Spect 308, 123757 (2024)
M. Monisha, G. NirmalMazumder, Soumen Mandal Lakshminarayana, Sudha D. Kamath, Energy transfer and luminescence study of Dy3+ doped zinc-aluminoborosilicate glasses for white light emission. Ceram. Int. 47(1), 598–610 (2021). https://doi.org/10.1016/j.ceramint.2020.08.167
P. Arun. Joseph, K. Maheshvaran. Jeganatha, I. Arul Rayappan, Structural and optical studies on Dy3+ ions doped alkali lead borophosphate glasses for white light applications. J Non-Cryst Solids 557, 120652 (2021)
Acknowledgements
We thank BRNS for the Project, entitled “Synthesis of novel Ln incorporated nonsilicate glass ceramics as radiation shielding materials,” No. 59/14/07/2020-BRNS/10096.
Author information
Authors and Affiliations
Corresponding author
Ethics declarations
Conflict of interest
The authors declare no competing interests.
Rights and permissions
Springer Nature or its licensor (e.g. a society or other partner) holds exclusive rights to this article under a publishing agreement with the author(s) or other rightsholder(s); author self-archiving of the accepted manuscript version of this article is solely governed by the terms of such publishing agreement and applicable law.
About this article
Cite this article
Deepa, P., Murugasen, P., Suresh, A.A. et al. Luminescence features of Dy3+-doped B2O3 and B2O3-P2O5 hosts for optical devices: the linear and non-linear optical studies. emergent mater. 7, 1715–1731 (2024). https://doi.org/10.1007/s42247-024-00696-z
Received:
Accepted:
Published:
Issue Date:
DOI: https://doi.org/10.1007/s42247-024-00696-z