Abstract
New Schiff base was synthesized from condensation of anthracene-10-carbaldehyde with 4-amino-3-mercapto-1,2,4-triazin-5(4H)-one. Resulted azomethine was characterized via elemental analysis, infrared and 1H-NMR spectroscopy. Thereafter, novel Schiff base complexes with Co(II), Ni(II), Zn(II) and Cu(II) metals were prepared and characterized by analytical, conductivity data, proton-NMR, IR, UV–Visible, ESR and fluorescence spectroscopy, magnetic moment as well as cyclic voltammetry. These physicochemical techniques suggest monobasic bidentate behaviour of ligand towards metal ions. Thermal stability and degradation kinetics have been studied using thermogravimetric techniques and some other thermal parameters are also reported. All the complexes exhibit octahedral geometry except copper complexes which show square planar arrangement around the metal centre. Low conductivity data of all compounds reveal their neutral and non-electrolytic character. Antimicrobial potentials of Schiff base and its metal chelates were evaluated against wide spectrum of bacterial cultures such as P. aeruginosa, S. aureus, B. subtilis, E. coli and fungi like A. niger and A. flavus.
Similar content being viewed by others
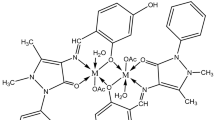
Avoid common mistakes on your manuscript.
Introduction
4-Amino-3-mercapto-1,2,4-triazin-5(4H)-one derivatives have been studied due to their herbicidal, antimicrobial and anticancer activities [1]. Many transition metal chelates of Schiff bases having 4-amino-3-mercapto-6-methyl-1,2,4-triazin-5(4H)-one have been reported [2,3,4,5]. But less work has been done on 4-amino-3-mercapto-1,2,4-triazin-5(4H)-one based metal chelates. This kind of compounds have many applications in medicinal, catalytic and industrial field [6, 7]. In coordination chemistry, the chemistry of transition metal complexes having heterocyclic moieties with thione/thiol groups has been an interesting research area in synthetic inorganic chemistry due to their diverse structural features and importance in biological field also.
Anthracene owns an exclusive electron-rich assembly with small electronic bandgap and shows intense blue fluorescence. Thus, it has been employed as a production fragment in diverse emissive objects [8, 9]. Anthracene derivatives are one of the promising structures due to their intensive luminescence properties and high thermal stabilities. Interesting fluorescent properties are revealed by anthracene derivatives [10]. The emission of anthracene derivatives lies in the visible region due to its intermolecular packing and conjugation over the extended area of the aromatic ring. Such conjugated organic moieties having some sort of optoelectronic properties are still of interest in spite of commercialization of such materials. Among such compounds, Schiff bases have been successful in grabbing the attention of researchers. Derivatives of Schiff bases having fluorescent chromophore can be utilized as sensors for metal ion centres. The fluorescent properties and quantum yields of metal complexes get changed with respect to their parent ligand after complexation with different metal ions [11].
Medicinal inorganic chemistry has become of great interest since last few decades. Resistance of microbes towards commonly existing antibiotics is causing problems which directs the inorganic researchers to synthesize new antimicrobial compounds to combat these obstinate pathogens. Schiff bases having heterocyclic aromatic moieties have been proved proficient precursors for synthesizing novel compounds of biological importance. Metal chelates of biologically active Schiff bases have been found more efficacious than free ligands. Metal complexes of transition metal ions can exhibit amazing pharmacological activities like antimicrobial, antiproliferative, antidiabetic, anti-inflammatory and anticancer while such potential is not noticed when the ligand or inorganic metallic salts are used individually [12,13,14,15]. Most appropriate metals for aforementioned purpose are transition metals because transition metals can exhibit different geometries and coordination numbers along with variable oxidation states as compared to other main group metals. Nickel is found in active sites of many enzymes like hydrogenases and CO-dehydrogenases. In its common oxidation state of + 2, numerous nickel complexes have shown their importance in antimicrobial and antiproliferative activities [16]. Zinc metal possesses crucial position in metabolism, immune response, functioning of liver and strengthening of bones being a part of enzymes for example alkaline phosphate enzyme, superoxide dismutase, alcohol dehydrogenase and protein kinase-C. Cu(II) and Zn(II) metal composites have been the subject of vast research work due to their excellent fluorescent and antimicrobial properties [17,18,19,20,21,22,23,24,25]. Cobalt, copper, zinc and nickel metals are well-recognized elements in our biological system. Also the antimicrobial potential of many drugs can be boosted after chelation with transition metals. So it becomes essential to know more about the bonding of ligands with metals and their structural properties to develop operative antimicrobial metal complexes.
The present research comprises of the design and synthesis of a fresh series of transition metal complexes of azomethine being condensation product of anthracene-10-carbaldehyde with 4-amino-3-mercapto-1,2,4-triazin-5(4H)-one. Their properties were investigated experimentally by using UV–visible, proton-NMR, IR, fluorescence, ESR spectroscopic techniques and cyclic voltammetry. Additionally, thermal profiles of the metal complexes were examined by thermogravimetric analysis. The bioactivities like antimicrobial and antifungal of all synthesized compounds have been reported here.
Experimental section
Materials and methods
Chemicals
All chemicals were of reagent grade. Acetate salts of Co(II), Ni(II), Cu(II), Zn(II) and anthracene-10-carbaldehyde were obtained from Sigma-Aldrich and used as supplied. Solvents such as dimethylformamide (DMF), dimethyl sulphoxide (DMSO), ethanol, acetic acid, diethylether and methanol were of analytical grade and used as received without further purification.
Instrumentation
Infrared (IR) spectra of the pure compounds were acquired on a MB3000 ABB spectrophotometer in 4000–30 cm−1 range using KBr pellets. 1H-NMR spectra of the synthesized free ligand and zinc complexes were obtained in deuterated DMSO using tetramethylsilane (TMS) as internal standard on a JEOL-400 NMR spectrometer. Thermo Scientific flash 2000 CHN Elemental Analyzer was adopted for C, H and N analyses of compounds at central instrumentation laboratory (CIL), Punjab University, Chandigarh. Estimation of nickel, zinc and copper metal ions was carried out by gravimetric methods, and cobalt was estimated by titration with 0.01 M EDTA [26]. Mass spectra were recorded on Waters Xevo G2-SX QTOF spectrophotometer. Electronic data of ligand and its complexes were obtained on T-90 (PG instruments Ltd.) UV–visible spectrophotometer in 900–190 nm range. EPR spectral information of copper samples were obtained using Varian E112 X-Band Spectrophotometer employing at 9.097 GHz frequency and field was calibrated with 2,2-Diphenyl-1-picrylhydrazyl (DPPH) at the SAIF, IIT Bombay. Magnetic properties of the samples were recorded on a vibrating sample magnetometer of model PAR-155, in which Hg[Co(CNS)4] was used as calibrant. Thin layer chromatographic plates were used to check the purity of ligand and metal chelates. Molar conductance of the complexes in DMF solution was measured using Systronics conductivity bridge. The Perfit electrical melting point apparatus was employed for recording melting points. The fluorescence spectra of all samples were monitored on SHIMADZU RF-5301 PC spectrofluorometer in dimethylformamide solution. Thermogravimetric analysis of metal complexes was achieved under nitrogen atmosphere at a heating rate of 10 °C per minute at Central Instrumentation Facility (CIF), LPU, Phagwara. Ivium Stat Electrochemical Analyzer was employed to perform electrochemical study of compounds using tertbutyl ammonium perchlorate as a supporting salt.
Biological activity investigation
Agar well diffusion method was applied for antibacterial examination as described in our former research paper [4]. The antibacterial activities of synthesized samples have been checked with regard to two Gram-positive bacteria (Staphylococcus aureus MTCC-96, Bacillus subtilis MTCC-121) and two Gram-negative bacteria (Escherichia coli MTCC-1652, Candida albicans MTCC-183) on nutrient agar medium. S. aureus is a member of microbiota of the body and possesses thick peptidoglycan layer. It causes joint infections, pneumonia, skin infections like boils and cellulitis. B. subtilis is non-pathogenic, Gram-positive bacteria. Although, it is one of the most commonly used bacteria for the production of enzymes still B. subtilis causes endocarditis, pneumonia and septicemia. However, these infections were noted in patients having very low immunity. E. coli are non-sporing with thin cell walls and lower intestine of warm-blooded animals is their primary habitat. These can cause various diseases like intestinal infections for e.g. UTIs, sepsis, meningitis, etc. C. albicans lies in category of pathogenic yeast, found in mouth and gastrointestinal tract of adults. It is one of the frequently isolated organisms involved in bloodstream infections. A. flavus is the most widely distributed fungi accountable for aflatoxin contamination of foodstuffs. It is also known for the production cancer-causing groups of mycotoxins. Aspergillus niger is a food spoilage fungus. It is one of the major causes of ‘black mould’ that is found on the surfaces of certain foods.
The antifungal activities were checked against two fungal types (Aspergillus niger MTCC-872 and Aspergillus flavus MTCC-873) by poisoned food technique. Solutions of compounds were prepared in dimethyl sulphoxide (DMSO). Agar medium was shifted to petri plates and plates were swabbed with 100 μL inocula of test microorganisms and kept for 15–20 min for adsorption. Using a sterile cork borer of 8 mm diameter, wells were bored in medium of agar plates, already seeded with the test microorganisms. 200 µL of every sample was poured in each well. The agar plates were preserved at 4 °C for minimum 30 min to let the dispersal of the sample to agar channel. The plates were then incubated at 37 °C for bacteria and 30 °C for fungi, respectively. The diameters of inhibition zones were specified after 24 h and 7 days for bacteria and fungi, respectively, taking the consideration of the control values (DMSO). Diameters of fungal colonies were determined as mycelial inhibition percentage.
Where dc is mean diameter of fungal colony in DMSO control and dt is mean diameter of fungal colony in experimental samples. Each experiment was performed in triplicates. Ciprofloxacin and Fluconazole were utilized as standard drugs with respect to bacteria and fungi separately. The minimal concentration of all samples which hindered the growth of bacteria was also checked and reported as minimum inhibitory concentration (MIC).
Syntheses
Synthesis of 4-(anthracen-10-ylmethyleneamino)-3-mercapto-1,2,4-triazin-5(4H)-one (Scheme 1)
4-amino-3-mercapto-1,2,4-triazin-5(4H)-one (AMTO) was prepared using reported literature [27]. Equimolar ethanolic solutions of AMTO (1.58 g, 11 mmol) and anthracene-10-carbaldehyde (2.27 g, 11 mmol) containing few drops of acetic acid were mixed and maintained under reflux for 90 min. Crystalline yellow solid was recovered after cooling the reaction mixture. The product was separated by filtration, washed with cold ethanol and diethylether and recrystallized to obtain pure ligand corresponding to 81% yield. Scheme given below illustrates the formation of ligand.
Synthesis of 1:1 transition metal complexes
Hot stirred ethanolic solutions of the ligand (0.298 g, 0.9 mmol, 20 mL) and acetates of cobalt (0.224 g, 0.9 mmol), nickel (0.224 g, 0.9 mmol), copper (0.180 g, 0.9 mmol) and zinc (0.198 g, 0.9 mmol) were mixed gradually corresponding to 1:1 (M:L) molar ratio. The mixtures were maintained under reflux with stirring nearly for 2 h. Resulting coloured precipitates were filtered out and washed with small portions of ethanol, diethyl ether and then kept in a desiccator in the presence of anhydrous CaCl2.
Synthesis of 1:2 transition metal complexes
For preparation of 1:2 (M:L) metal complexes, ethanolic solutions of above stated amounts of metal acetates of cobalt, nickel, copper and zinc were reacted with heated ethanolic solutions of ligand (0.596 g, 1.8 mmol). Then reaction mixtures had been refluxed for about 2 h. Coloured solid products were filtered out, washed with small portions of ethanol and diethyl ether. Final products were placed in a desiccator over anhydrous CaCl2.
Results and discussion
The ligand and metal complexes are coloured, air-stable, non-water absorbing and get decomposed at higher temperature. The metal complexes are soluble in DMSO, DMF and acetone and not soluble in other conventional organic solvents. The molar conductance values of metal complexes in DMF (10–4 M) are in the range of 10.24–21.40 \(\Omega\)−1 cm2 mol−1, such low conductance values suggest non-electrolytic behaviour of metal complexes. Some physical and elemental analysis data are depicted in Table 1.
IR spectral studies
Bonding of metal ions to the ligand may be determined by assessing IR spectra of the metal complexes and ligand. So, the ligand and its metal complexes have been characterized with IR spectral studies (Table 2). The further deductions can be drawn: The infrared spectrum of ligand exhibits a strong band at 1636 cm−1 due to υ (C=O) of triazine part. No significant change in the carbonyl band position in the IR spectra of the metal complexes, designates that the carbonyl group in these complexes is not involved in coordination to the metal ions [28]. A medium intensity band observed in IR spectrum of ligand at 1589 cm−1 is assigned to ν(CH=N) azomethine group which gets shifted to lower wavenumber, 1561–1582 cm−1 in the IR spectra of metal complexes indicating the coordination of azomethine nitrogen to metal ions [29]. The double bond character of the azomethine group decreases due to the attachment of nitrogen atoms to the metal ions and hence corresponding band gets shifted. These results are consistent with the outcomes obtained from similar structured compounds documented by researchers [30]. New absorption bands in low frequency regions 458–484 cm−1 and 338–342 cm−1 are observed due to ν(M–N) and ν(M-S), respectively [31]. A weak band at 2700 cm−1 is observed due to ν(SH) in the spectrum of Schiff base which gets disappeared on complexation, indicating deprotonation of thiol group and coordination through sulphur [32]. In the IR spectra of 1:1 metal complexes, characteristic band in region 1740–1744 cm−1 was attributed to ν(OAc). Asymmetric νas(OAc) and symmetric νs(OAc) stretches in metal complexes occur at about 1591–1594 cm−1 and 1373–1388 cm−1, respectively, with wavenumber separation of ∆ν = 203–221 cm−1 indicating monodentate nature of acetate group [33]. The broad bands were emerged in the region 3209–3310 cm−1 due to ν(OH/H2O) vibrations of coordinated water molecules in IR spectra of all the complexes except in 1:2 Cu(II) metal complex [34]. Further existence of water molecules in coordination spheres of compounds was endorsed by thermogravimetric studies.
1H-NMR spectroscopy
1H-NMR spectra of HL and its zinc metal chelates were recorded in DMSO-d6 (Figs. S2-S4 in supporting information) and data are presented in Table 3. A singlet was observed at δ 8.92 ppm due to azomethine proton (–CH=N) in the ligand which got shifted to δ 8.96 ppm and δ 8.95 ppm in 1:1 and 1:2 Zn complexes, respectively, clearly indicating coordination of azomethine nitrogen to zinc ions [35]. Another singlet at δ 14.15 ppm corresponding to thiol proton (–SH) in ligand’s spectrum got disappeared in spectra of Zn(II) complexes propounding deprotonation of thiol group and further coordination of sulphur to zinc metal ions [36]. In the 1H-NMR spectrum of ligand (supporting information Fig. S1), aromatic protons Ha and Hd exhibit doublet at 8.91 and 8.24 ppm, respectively, with 8.4 Hz coupling constant. Two multiplets are observed in the range 7.73–7.69 ppm and 7.66–7.63 ppm corresponded to Hb and Hc protons of anthracene moiety. A singlet at 8.06 ppm in ligand’s spectrum, is due to aromatic proton of triazine ring. Signals at 4.32 ppm and 4.22 ppm are due to coordinated water molecules in 1:1 and 1:2 Zn complexes, respectively. The signal due to acetate group is only observed in 1:1 Zn compound at 1.31 ppm. Obtained 1H-NMR data of ligand and zinc metal complexes were also compared to that of similar structured compounds. The results reveal that signal due to azomethine proton got shifted in complexes and signals due to phenol/thiol hydrogen are disappeared in case of metal complexes which confirm the binding of O− or S− to the metal centres [25].
Mass spectral studies
Formulation of ligand and its metal chelates has been determined from elemental analytical data, IR and NMR spectroscopy which is further supported by mass spectrometry. Mass spectra of some samples showed molecular ion peaks at m/Z 332.13, 505.39, 504.52, 726.04, 763.88 correspond to their molecular formulas C18H12N4OS, C20H20N4O6SCo, C20H20N4O6SNi, C36H22N8O2S2Cu, C36H26N8O4S2Zn, respectively. These results support ML type stoichiometry in 1:1 cobalt and nickel metal complexes and ML2 type stoichiometry in 1:2 copper and zinc metal complexes. The series of peaks other than molecular ion peaks are indicative of various fragments in samples and intensity of each peak suggests its stability among all fragments [37]. A good agreement between suggested molecular formula by elemental analysis and molecular ion peaks by mass spectral data is observed. Mass spectra of compounds are shown in Figs. S5-S9 (Supporting information).
Magnetic moment measurements and electronic spectral studies
The magnetic moment measurements along with electronic spectral data afforded much knowledge concerning the arrangement of the ligand around the metal centres which is represented in Table 4. Absorption spectra of all compounds were noted in solvent DMF with concentration 50 μm at room temperature. Ligand showed two intense maxima in its spectrum at 36,101 cm−1 and 28,328 cm−1 owing to π–π* and n–π* transitions. Bathochromic shifts in these transitions were noted in metal complexes which endorses metal–ligand coordination in chelates.
Cobalt complexes
Both Co(II) complexes showed magnetic moment 4.53–4.62 BM, which is properly inside the anticipated range of octahedral complex, i.e. 4.3–5.2 BM. Co(II) complexes display two absorption bands in region 10,101–10,351 cm−1 (ν1) and 20,920–21,186 cm−1 (ν3) assigned to spin allowed 4T1g → 4T2g(F) and 4T1g → 4T1g(P) transitions, respectively, which signify distinctive bands shown by octahedral Co(II) complexes of high spin [38]. ν2 value is not observed in electronic spectra but it has been calculated by using relation ν2 = ν1 + 10Dq. Ligand field factors like crystal field stabilizing energy, Racah parameter (B), nephelauxetic ratio (β) and β% have also been computed for these complexes with Band-fitting equation [39]. The crystal field stabilizing energy (Dq) values were located at 1133.6 and 1160 cm−1. These values properly lie in the range shown by octahedral compounds. The interionic repulsion parameter (Racah parameter), i.e. B, is found to be 776.3–803.5 cm−1 implying a generous overlapping of ligand and metal orbitals. The value of β (nephelauxetic ratio) below unity advises partial covalency in the metal ligand bond in these complexes [40].
Nickel complexes
Nickel complexes, with magnetic moment in the range 3.29–3.47 BM exhibit octahedral atmosphere around the central metal. Nickel(II) complexes mostly show three absorption bands for spin allowed d–d transitions corresponding to 3A2g → 3T2g (F) (ν1), 3A2g → 3T1g (F) (ν2) and 3A2g → 3T1g (P) (ν3) transitions which are compatible with octahedral environment around nickel centres. Here, both 1: 1 and 1: 2 Ni(II) complexes of HL show above-mentioned three transitions in region 10,362–10,373 cm−1 (ν1), 17,035–17,094 cm−1 (ν2) and 24,038–24,154 cm−1 (ν3) signifying distorted octahedral geometry for the complexes [41]. The ratio ν2/ν1 (1.64–1.65) supported the octahedral environment around the central metal ion. Other important crystal field parameters (β%, Dq, β, B) were also computed for these compounds which reveal covalent nature of nickel-ligand bonds [42].
Copper complexes
Cu(II) complexes have 1.91– 2.02 BM magnetic moment values which fall in the normal range (1.7–2.2 BM) for square planar geometry. The electronic spectra of Cu(II) complexes showed broad absorption band at about 17,605–17,793 cm−1 which is attributed to overlapped transitions, i.e. 2B1g → 2A1g and 2B1g → 2B2g [43]. It is a representative band of square planar geometry around the Cu(II) centre [4, 44].
Electron spin resonance study
To know more about type of bonding between metal and Schiff base, ESR analysis of copper complexes was performed. ESR spectra of copper metal complexes were monitored in magnetic field strength of 0.3 T at liquid nitrogen temperature, on X-band at 9.097 GHz using TCNE as marker. ESR studies also give information about location of unpaired electron in different orbitals. The values of g-tensor are observed in order: \(g_{\parallel } \left( {2.26} \right) > g_{ \bot } \left( {2.10} \right) > 2.0023\) for C20H16N4O4SCu and \(g_{\parallel } \left( {2.27} \right) > g_{ \bot } \left( {2.07} \right) > 2.0023\) for C36H22N8O2S2Cu complex. Experimental data indicate that single electron rests in \(d_{{x^{2} - y^{2} }}\) orbital primarily and 2B1g can be designated as ground state. This observation also commended square planar geometry of both complexes [31]. Exchange interaction parameter (G) was computed from equation \(G = \frac{{g_{\parallel } - 2.0023}}{{g_{ \bot } - 2.0023}}\). Exchange interaction is negligible if G > 4 and extensive exchange interaction is found in the metal complexes if it is smaller than 4. The G values are found less than 4, confirming exchange interactions amid Cu(II) centres [45]. Deviation of gav from 2.0023 (ge) indicates covalent character of metal–ligand bonds in both copper complexes. The \(g_{\parallel } < 2.3\) also confirm covalent M-L linkages. The empirical factor, \(f = g_{\parallel } /A_{\parallel }\), is an indicator of deformation and varies from 105–135 cm−1 for minor to major distortion in square planar geometries moreover values lower than 135 cm−1 are observed for square planar structures. The f values for 1:1 and 1:2 copper complexes are 82.48 and 93.03, respectively, supporting square-planar geometry. ESR spectrum of C20H16N4O4SCu is displayed in Fig. 1. Molecular orbital coefficients, α2 (covalent in-plane sigma bonding), β2 (covalent in-plane pi-bonding) and γ2 (out of plane pi-bonding), were calculated to better define the nature of M-L bonds, using following equations.
The 0.5 value of α2 directs ample covalent bonding, while 1.0 value advises ionic bonding. Since α2 values for invested copper complexes lie near ~ 1.0 which is anticipated for partial ionic nature of the bonds [46]. Observed β2 values (0.62 and 0.70) are less than unity and also less than α2 values which indicate the presence of more in-plane π–bonding than in-plane σ‐bonding in metal complexes. Values of \(A_{\parallel }\) for copper complexes are found greater than 140 × 10–4 cm−1, i.e. 274 × 10–4 and 244 × 10–4 cm−1, which further supports square planar arrangement around copper centres in both complexes [47]. According to Hathaway and Billing, for pure sigma bonding, the relation between orbital reduction factors is \(K_{\parallel } > K_{ \bot } \approx\) 0.77, \(K_{\parallel } < K_{ \bot }\) for in-plane π–bonding while \(K_{\parallel } > K_{ \bot }\) for out of plane π–bonding. The equations are:
Examined \(K_{\parallel }\) (0.68, 0.71) and \(K_{ \bot }\) (1.03, 0.73) values for both complexes imply larger impact of in-plane pi-bonding than out of plane pi-bonding in M-L linkages. Also, greater \(K_{ \bot }\) values than \(K_{\parallel }\) indicate the presence of significant in-plane π–bonding which supports the results obtained from molecular orbital parameters.
Redox properties
Cyclic voltammograms of copper chelates were monitored in DMF containing 0.1 M of tetrabutylammonium perchlorate electrolyte with a scan rate of 0.01 Vs−1 in a potential range − 1.5 V to + 1.0 V to enquire their redox properties. In recorded voltammogram of 1:1 Cu complex, two peaks are observed at Epc = 0.34 V and Epc’ = − 0.45 V assigned for the reactions Cu(II) to Cu(I) and Cu(I) to Cu(0) independently on the reduction side and likewise two peaks associated with Cu(0) to Cu(I) and Cu(I) to Cu(II) are observed at Epa = − 0.18 V and Epa’ = 0.60 V on the oxidation side. These couples are quasi-reversible with high values of ∆Ep, i.e. 260 mV and 270 mV. 1:2 Cu complex exhibits two redox couples; one is observed at Epc = 0.235 V on reduction side and at Epa = 0.36 V on oxidation side for Cu(II)/Cu(I) and another redox couple Cu(I)/Cu(0) is observed at Epc’ = − 0.44 V on reduction side and at Epa’ = − 0.245 V on oxidation side. Ratio of anodic to cathodic peak currents for redox couples lie in range 0.26–0.72 and 0.21–1.83 for 1:1 Cu complex and 1:2 Cu complex, respectively, which suggest non-equivalent current intensity and are consistent with quasi-reversible processes. ∆Ep = 125 mV and 195 mV values of redox couples of 1:2 Cu complex suggest quasi-reversible processes [48, 49]. When these cyclic voltammograms were compared with that of ligand, it was found that there is no oxidation and reduction peaks in CV of ligand. Cyclic voltammogram of copper complexes are exposed in Fig. 2.
Thermogravimetric analysis
Some of prepared metal complexes were examined for their thermal properties. Thermogravimetric curves of C36H26N8O4S2Co, C20H20N4O6SNi, C20H16N4O4SCu and C36H26N8O4S2Zn complexes are shown in Fig. 3. These complexes followed a similar pattern of decomposition. The degradation temperatures with decomposed moieties and mass loss percentages of these metal complexes along with their residues are illustrated in Table 5.
The complex C36H26N8O4S2Co decomposes in three different stages. First decomposition stage in temperature range 80–140 °C may be ascribed to loss of coordinated water molecules with a weight loss of 4.79% (calculated 4.75%). The second step of decomposition in temperature range 141–575 °C might attribute to loss of organic moiety with found weight loss 53.39% (calculated 53.84%) and the final stage of decomposition between temperature 576 °C and 920 ˚C corresponded to loss of triazine moiety with weight loss 28.66% (calculated 29.30%). After these degradation steps, final weight of residue corresponded to CoS [50, 51]. The compound C20H20N4O6SNi degrades in three steps. The first step owes in mass loss of 9.93% (calculated 10.73%) in temperature range 80–144 ˚C equivalent to weight loss of three H2O molecules. Then, organic part of molecule gets decomposed with mass loss of 48.75% (calculated 49.48%) between temperature range (145–600 ˚C). Last step involves a weight loss of 25.78% (calculated 24.84%) in the temperature range 601–956 ˚C due to decay of triazine moiety. C20H16N4O4SCu degrades in three stages. The first one seems to be sufficient to weight loss of coordinated water molecule and acetate group with found weight loss of 15.46% (calculated 16.31%) between 100 and 270 ˚C. In second stage, decomposition of organic moiety takes place from 271 to 720 ˚C with weight loss of 40.02% (calculated 40.25%). In the third step decomposition occurs between temperature 721 °C and 960 ˚C corresponded to loss of triazine moiety with a weight loss 27.04% (calculated 26.48%). C36H26N8O4S2Zn also shows three decomposition stages. In first stage, weight loss of 4.94% (calculated 4.71%) corresponds to loss of two coordinated water molecules in temperature range 80–130 ˚C. Second stage eliminates organic part of complex between 131 and 510 ˚C temperature with weight loss of 59.15% (calculated 58.10%) and in last step of decomposition triazine moieties are liberated with a weight loss of 26.51% (calculated 26.43%) in temperature range 511–960 ˚C.
Kinetic studies
Well-observed decomposition stages were selected to measure kinetic parameters of metal chelates. Activation energies of decomposition stages have been calculated by applying Coats and Redfern method [52]. Coats and Redfern equation is written as:
where T is temperature; A is pre-exponential factor (min−1); R is universal gas constant (8.314 J/K/mol), E is energy of activation (kJ/mol); β is heating rate (°C min−1); α is degree of conversion; g(α) is decomposition mechanism and \(g\left( \alpha \right) = \frac{1}{1 - \alpha } - 1\) (in case of second order reaction). Activation energy was obtained from the slope of the plot between \(ln\left[ {\frac{g\left( \alpha \right)}{{T^{2} }}} \right]\) and 1000/T. Apparent activation energies for different decomposition stages by applying Coats and Redfern method and analogous regression coefficient values (R2) are presented in Table 6 and graphs are displayed in Fig. 4. Activation energy values (E2) of major decomposition step followed the sequence Zn(II) > Cu(II) > Co(II) > Ni(II) complexes and suggested similar thermal stability order of these metal complexes [36].
Fluorescence spectroscopic studies
Metal complexes fluorescence studies are very important because of their applications such as in photochemistry, luminescent displays and as chemical sensors [11]. The photoluminescent properties of Schiff base and all metal chelates were investigated in DMF solvent at room temperature. The emission spectra were noted in 380–800 nm span of wavelength. The comparable fluorescence spectra of 1:1 and 1:2 metal complexes with Schiff base are presented in Fig. 5. Upon irradiation of UV-light, with excitation wavelength 370 nm, Schiff base exhibited weak fluorescence with respect to all metal complexes which are comparatively highly fluorescent active. Schiff base exhibits fluorescent emission bands 445 nm along with two shoulders at 418 nm and 474 nm which may be attributed to \(\pi - \pi^{*}\) intraligand transitions [53, 54]. The shapes of emission spectra of metal compounds closely resemble to that of their parent Schiff base. So, fluorescence may be assigned due to intraligand transitions or ligand to metal charge transfer processes. This type of fluorescence is called CHEF (Chelation enhancement of fluorescence). Emission intensity of all metal complexes is higher than that of ligand. The fluorescence quenching in case of ligand may be probably due to incident of PET (photoinduced electron transfer) action which is prevented after complexation. The enhancement in emission intensity is suggested because of complexation of ligand and metal ion centres. Rigidity of the ligand is increased after complexation which further causes to minimize the energy loss [19, 55]. These results indicate that the newly synthesized ligand might be appropriate to sense cobalt, nickel, copper and zinc ions. Both cobalt complexes show high intensity fluorescence bands at 449 nm. Although Ni(II) and Zn(II) complexes show maximum emission bands at 447 nm but Zn(II) complexes display more fluorescence intensity than Ni(II) complexes. 1:1 and 1:2 copper chelates flourish intense emission bands at 475 nm. All these complexes also show some shoulder bands along with main higher intensity emission bands. Among all compounds copper chelates are found to possess higher fluorescent properties.
Quantum yield calculations
Quantum yield is stated as the fraction of molecules of a sample that emit photons after excitation by the source. Uncertainties related to apparatus and samples can influence the quantum yield values. So, slit width during the whole experiment was kept constant at 5 mm in order to minimize the uncertainties, also the quinine sulphate (\(\varphi_{R} = 0.54)\) was selected as reference solution. Five different concentrations of each sample were prepared in DMF (\(\eta_{s} = 1.4305)\) whereas reference was dissolved in 0.1 M H2SO4 (\(\eta_{R} = 1.3333).\) Fluorescence spectra of all samples were recorded by keeping absorbance values below 0.5. Slopes were determined by plotting the graphs between different concentrations of samples and fluorescence intensity [56]. After knowing the slopes, quantum yields of ligand and metal complexes were calculated by well-known William’s method using the following formula:
where φ represents quantum yield, m values represent slopes of fluorescence intensity versus concentration curves of compounds and η is refractive index of solvent [57]. Subscripts R and S are taken for reference and sample, respectively. Calculated quantum yields are presented in Table 7. Quantum yield varies from 4.94 to 14.23% and copper complexes exhibit highest quantum yield among all prepared compounds.
CIE parameters
International commission on illumination (CIE) gave a set of parameters to estimate the colour of radiated light by a body. CIE coordinates are enumerated in Table 7. The colour coordinates of synthesized samples match well with bluish colour of National Television Standard Committee (NTSC: x = 0.14, y = 0.08) and European Broadcasting Union (EBU: x = 0.16, y = 0.07). CIE chromaticity diagrams (Fig. 6) were plotted from this data using colour calculator software. Observed colour coordinates and quantum yield values for copper complexes advocate their application in blue light emitting objects in several electroluminescent materials like OLEDs [58]. High quantum yield values of copper complexes also endorse above purpose.
Antimicrobial properties
The antibacterial and antifungal potential of synthesized samples was checked with respect to two Gram-positive, two Gram-negative, two fungal organisms as well as compared with standard drugs. The results have been shown in form of diameter of zone of inhibition in Table 8 and Fig. 7 for antibacterial screening and in Fig. 8 for mycelial % growth for antifungal screening. Solvent DMSO used in this study was also screened against all pathogens taken and did not exhibit any activity. Also, the metal salts used in this study did not exhibit any antibacterial potential against tested microorganisms. The compounds exhibited more activity against gram-positive bacteria than gram-negative bacteria. Pictorial representation of zone of inhibition of some of the metal complexes against these organisms are given in Fig. S12-S16 in supporting information.
A good antimicrobial molecule either effectively kills bacteria or slows down their speed of growth without being much toxic to tissues present in their close proximity. Such molecule may opt any of the two mechanisms; one is chemically interfering with synthesis of components of bacteria and other is to circumvent the mechanism of antimicrobial resistance. Antimicrobial agents can bind to the lipid-A component of lipopolysaccharide and cause alterations in structures, this will further result into fast destruction of bacterial cell membrane. Large hydrophobic moieties bind to the membrane proteins and change the permeability of the membranes.
The basis for this difference in sensitivity between compounds might be credited to the difference in morphological constitutions of these microorganisms [47]. This can be explained due to the presence of lipopolysaccharides in outer phospholipidic membrane of Gram-negative bacteria cell wall which act as diffusion barrier for compounds making them less susceptible [59]. On the other hand, the Gram-positive bacteria are more susceptible due to comprising of only outer peptidoglycan layer which is not an effectual permeability obstruction. In spite of this permeability differences, some samples still exerted some degree of inhibition against Gram-negative bacteria as well. Individual metal ion plays its role in the antibacterial activity of metal complexes. The inhibitory potential of some of the tested compounds was found to be good as compared to that of reference drugs. All these findings show that antimicrobial activity not only depends on molecular structure of bacterial strains but also on the molecular structure of the tested compounds. MIC values of compounds are also shown in Fig. 9 (Table T1 in supporting information). Both copper and zinc complexes exhibit better antibacterial property than other compounds and these complexes are also better fungicidal with mycelial cell growth in range 44–61% against A. niger and A. flavus organisms. Antimicrobial properties of synthesized complexes were compared with other similar complexes. Outcomes of comparable study show that these complexes possess better activity towards bacterial strains and zinc complexes are more effective [24, 30, 60]. Structural moieties and nature of the central metal of these complexes form the basis of activity of these complexes. Advised structures of all metal chelates are shown in Fig. 10.
Conclusion
In summary, this paper describes the synthesis and spectroscopic characterization of a series of Co(II), Ni(II), Cu(II) and Zn(II) complexes with new Schiff base ligand derived from condensation of anthracene-10-carbaldehyde with 4-amino-3-mercapto-1,2,4-triazin-5(4H)-one. All the synthesized compounds have been characterized with the help of various physiochemical techniques. Thermal analysis and infrared spectral studies confirmed the presence of water molecules in coordination sphere of complexes. Thermal stability and kinetic factors were computed using Coats–Redfern method. Copper complexes are found to possess significant fluorescent properties with high quantum yield which endorse their uses in electroluminescent materials. Electrochemical studies of copper complexes indicate their redox behaviour. On the basis of analytical and spectral characterization, copper complexes are found to exhibit square planar structures and cobalt, nickel and zinc complexes are advised with octahedral geometries. All synthesized compounds have been investigated for antibacterial and antifungal activities. The inhibitory potential of some of tested compounds was found to be good against some organisms. Zinc metal chelates have been found with more inducing ability to constrain the growth of bacterial and fungal species than other metal complexes and ligand.
References
N.A.H. Alshammari, D.A. Bakhotmah, Polycycl. Aromat. Compd. (2022). https://doi.org/10.1080/10406638.2022.2025863
H.M. Diab, W.M.I. Hassan, I.A. Abdelhamid, A.H.M. Elwahy, J. Mol. Struct. (2019). https://doi.org/10.1016/j.molstruc.2019.07.047
B. Ramachandra, B. Narayana, Indian J. Chem. 38, 1297 (1999)
K. Singh, P. Turk, A. Dhanda, Eur. Chem. Bull. (2018). https://doi.org/10.17628/ecb.2018.7.194-202
K. Singh, R. Thakur, V. Kumar, Beni-Suef Univ. J. Basic. Appl. Sci. (2016). https://doi.org/10.1016/j.bjbas.2016.02.001
M.A. Ibrahim, R.M. Abdel-rahman, A.M. Abdel-halim, S.S. Ibrahim, H.A. Allimony, ARKIVOC 16, 202–215 (2008)
T.E. Ali, Eur. J. Med. Chem. (2009). https://doi.org/10.1016/j.ejmech.2009.06.022
Z. Zhang, Y. Zhang, D. Yao, H. Bi, I. Javed, Y. Fan, H. Zhang, Y. Wang, Crystal Growth Des. (2009). https://doi.org/10.1021/cg9008569
M. Barwiołek, M. Babinska, A. Kozakiewicz, A. Wojtczak, E. Szłyk, Polhedron (2017). https://doi.org/10.1016/j.poly.2017.06.019
D. Sek, M. Siwy, M. Grucela, G. Małecki, E.M. Nowak, G. Lewinska, J. Santera, K. Laba, M. Lapkowski, S. Kotowicz, E. Schab-Balcerzak, Spectrochim. Acta Part A Mol. Biomol. Spectrosc. (2017). https://doi.org/10.1016/j.saa.2016.12.013
R. Kalarani, M. Sankarganesh, G.G.V. Kumar, M. Kalanithi, J. Mol. Struct. (2020). https://doi.org/10.1016/j.molstruc.2020.127725
Z. Kazemi, Z. Chavoshpour-natanzi, V. Mirkhani, Inorganica Chim. Acta. (2020). https://doi.org/10.1016/j.ica.2020.120004
H. Kargar, R. Behjatmanesh-ardakani, V. Torabi, M. Kashani, Polyhedron (2021). https://doi.org/10.1016/j.poly.2020.114988
H. Kargar, V. Torabi, A. Akbari, R. Behjatmanesh, A. Muhammad, N. Tahir, J. Iran. Chem. Soc. (2019). https://doi.org/10.1007/s13738-018-01583-1
H. Kargar, F. Aghaei-meybodi, M.R. Elahifard, M.N. Tahir, M. Ashfaq, K. Shahzad, J. Coord. Chem. (2021). https://doi.org/10.1080/00958972.2021.1900831
M. Fallah-mehrjardi, H. Kargar, R. Behjatmanesh-ardakani, J. Mol. Struct. (2022). https://doi.org/10.1016/j.molstruc.2021.132037
N.K. Gondia, S.K. Sharma, Opt. Quantum Electron. (2017). https://doi.org/10.1007/s11082-017-1138-9
D. Majumdar, D. Das, S.S. Sreejith, S. Das, J. Kumar, Inorganica Chim. Acta (2019). https://doi.org/10.1016/j.ica.2019.02.022
Y. Pan, Y. Zhang, L. Wang, Appl. Organomet. Chem. (2020). https://doi.org/10.1002/aoc.5441
H. Kargar, A. Adabi, M. Nawaz, M. Ashfaq, K. Shahzad, J. Mol. Struct. (2021). https://doi.org/10.1016/j.molstruc.2021.130112
H. Kargar, A. Adabi, A. Khurram, S. Munawar, M. Ashfaq, J. Iran. Chem. Soc. (2021). https://doi.org/10.1007/s13738-021-02207-x
H. Kargar, F. Aghaei-meybodi, R. Behjatmanesh-ardakani, J. Mol. Struct. (2021). https://doi.org/10.1016/j.molstruc.2021.129908
H. Kargar, A. Adabi, M. Nawaz, M. Ashfaq, K. Shahzad, J. Mol. Struct. (2021). https://doi.org/10.1016/j.molstruc.2020.129842
H. Kargar, M. Fallah-mehrjardi, R. Behjatmanesh-ardakani, H. Amiri, A. Adabi, S. Sedighi-khavidak, K. Shahzad, M. Ashfaq, M. Nawaz, Inorganica Chim. Acta (2022). https://doi.org/10.1016/j.ica.2021.120677
H. Kargar, M. Fallah-mehrjardi, M. Ashfaq, K. Shahzad, M.N. Tahir, R. Behjatmanesh-ardakani, A. Rudbari, A.A. Ardakani, S. Sedighi-khavidak, J. Coord. Chem. (2021). https://doi.org/10.1080/00958972.2021.1990271
M. Heidelberger, H.P. Treffers, J. Gen. Physiol. (1942). https://doi.org/10.1085/jgp.25.4.523
A.S. Ramasubramanian, B. Ramachandra Bhat, R. Dileep, J. Rasayan, Chem 3, 122 (2010)
C.J. Dhanaraj, M.S. Nair, J. Coord. Chem. (2009). https://doi.org/10.1080/00958970903191142
A.A. Pawanoji, B.H. Mehta, Asian J. Chem. 21, 6869 (2009)
A. Sahraei, H. Kargar, M. Hakimi, M.N. Tahir, J. Mol. Struct. (2017). https://doi.org/10.1016/j.molstruc.2017.08.022
A. Gubendran, G. Gangatharan, V. Kumar, M. Palsamy, K. Gurusamy, R. Periakaruppan, Appl. Organomet. Chem. (2017). https://doi.org/10.1002/aoc.4128
K. Singh, D.P. Singh, M. SinghBarwa, P. Tyagi, Y. Mirza, J. Enzyme, Inhib. Med. Chem. (2006). https://doi.org/10.1080/14756360600838648
C.C.R. Sutton, G. Da Silva, G.V. Franks, Chem. A Eur. J. (2015). https://doi.org/10.1002/chem.201406516
K. Singh, S. Raparia, P. Surain, Med. Chem. Res. (2015). https://doi.org/10.1007/s00044-014-1298-0
S.H. Sumrra, M. Ibrahim, S. Ambreen, M. Imran, M. Danish, F.S. Rehmani, Bioinorg. Chem. Appl. (2014). https://doi.org/10.1155/2014/812924
K. Singh, P. Turk, A. Dhanda, Appl. Organomet. Chem. (2021). https://doi.org/10.1002/aoc.6088
A.A. Ardakani, H. Kargar, N. Feizi, M.N. Tahir, J. Iran. Chem. Soc. (2018). https://doi.org/10.1007/s13738-018-1347-6
A.S. Munde, A.N. Jagdale, S.M. Jadhav, T.K. Chondhekar, J. Serbian Chem. Soc. (2010). https://doi.org/10.2298/JSC090408009M
B.J. Hathaway, D.E. Billing, Coord. Chem. Rev. (1970). https://doi.org/10.1016/S0010-8545(00)80135-6
M.B. Fugu, N.P. Ndahi, B.B. Paul, A.N. Mustapha, J. Chem. Pharm. Res. 5, 22 (2013)
B. Geeta, K. Shravankumar, P.M. Reddy, E. Ravikrishna, M. Sarangapani, K.K. Reddy, V. Ravinder, Spectrochim. Acta Part A Mol. Biomol. Spectrosc. (2010). https://doi.org/10.1016/j.saa.2010.08.004
N. Kavitha, P.V. AnanthaLakshmi, J. Saudi, Chem. Soc. (2017). https://doi.org/10.1016/j.jscs.2015.01.003
A.B.P. Lever, D. Ogden, Inorg. Phys. Theor. 2041, 2 (1965)
G. Lupașcu, E. Pahonțu, S. Shova, ȘF. Bărbuceanu, M. Badea, C. Paraschivescu, J. Neamțu, M. Dinu, R.V. Ancuceanu, D. Drăgănescu, C.E. Dinu-Pîrvu, Appl. Organomet. Chem. (2021). https://doi.org/10.1002/aoc.6149
M.P. Kesavan, G.G. VinothKumar, J. DhaveethuRaja, K. Anitha, S. Karthikeyan, J. Rajesh, J. Photochem Photobiol B Biol (2017). https://doi.org/10.1016/j.jphotobiol.2016.11.024
Y. Abdi, N. Bensouilah, D. Siziani, M. Hamdi, A.M.S. Silva, B. Boutemeur-Kheddis, J. Mol. Struct. (2020). https://doi.org/10.1016/j.molstruc.2019.127307
M. Gaber, N. El-Wakiel, K. El-Baradie, S. Hafez, J. Iran. Chem. Soc. (2019). https://doi.org/10.1007/s13738-018-1494-9
A. Abayneh, T. Gebretsadik, S. Tadesse, M. Thomas, Adv. Chem. Engineer. Sci. (2018). https://doi.org/10.4236/aces.2018.84017
W. Benabid, K. Ouari, S. Bendia, R. Bourzami, M.A. Ali, J. Mol. Struct. (2020). https://doi.org/10.1016/j.molstruc.2019.127313
N. Özpozan, H. Arslan, T. Özpozan, M. Merdivan, N. Külcü, J. Therm. Anal. Calorim. (2000). https://doi.org/10.1023/A:1010171230450
S.M. Emam, D.A. Tolan, A.M. El-Nahas, Appl. Organomet. Chem. (2020). https://doi.org/10.1002/aoc.5591
S.M. Alshehri, A. Al-Fawaz, T. Ahamad, J. Anal. Appl. Pyrolysis. (2013). https://doi.org/10.1016/j.jaap.2013.01.004
M. Serhan, M. Sprowls, D. Jackemeyer, M. Long, I.D. Perez, W. Maret, N. Tao, E. Forzani, AIChE Annu., Meet. Conf. Proc. (2019). https://doi.org/10.1039/x0xx00000x
R.D. Costa, E. Ortí, H.J. Bolink, F. Monti, G. Accorsi, N. Armaroli, Angew. Chemie - Int. Ed. (2012). https://doi.org/10.1002/anie.201201471
S. Densil, C. Huei, C. Chia, L. Chen, A. Mathavan, V. Sathish, P. Thanasekaran, A. Ramdass, W.S. Li, Luminiscence (2018). https://doi.org/10.1002/bio.3477
N.K. Gondia, S.K. Sharma, Mater. Chem. Phys. (2019). https://doi.org/10.1016/j.matchemphys.2018.12.014
B. Brandner, Implementation of a Comparative Method for Measuring Photoluminescence Quantum Yields of Novel Compounds in Solution. (Oregon State University, 2016) https://ir.library.oregonstate.edu/concern/undergraduate_thesis_or_projects/8336h352k. Accessed 21 July 2017
L. Subha, C. Balakrishnan, S. Natarajan, M. Theetharappan, B. Subramanian, M.A. Neelakantan, Spectrochim. Acta A Mol. Biomol. Spectrosc. (2015). https://doi.org/10.1016/j.saa.2015.08.033
S. Yordanova, H.T. Temiz, I.H. Boyaci, S. Stoyanov, E. Vasileva-Tonkova, A. Asiri, I. Grabchev, J. Mol. Struct. (2015). https://doi.org/10.1016/j.molstruc.2015.08.012
A. Sahraei, H. Kargar, M. Hakimi, M. Nawaz, Transit. Met. Chem. (2017). https://doi.org/10.1007/s11243-017-0152-x
Acknowledgements
We express genuine gratefulness to DST-FIST programme 2017 and Department of Chemistry, Kurukshetra University, Kurukshetra, for providing essential research facilities.
Author information
Authors and Affiliations
Corresponding author
Supplementary Information
Below is the link to the electronic supplementary material.
Rights and permissions
About this article
Cite this article
Turk, P., Singh, K. & Dhanda, A. Synthesis, spectroscopic, electrochemical, thermal and antimicrobial studies of Ni(II), Zn(II), Cu(II) and Co(II) metal complexes of novel bidentate schiff base ligand. J IRAN CHEM SOC 19, 3797–3813 (2022). https://doi.org/10.1007/s13738-022-02567-y
Received:
Accepted:
Published:
Issue Date:
DOI: https://doi.org/10.1007/s13738-022-02567-y