Abstract
The study aimed at the development of elephant foot yam starch (EFYS) based edible film through blending of Xanthan (XG) and agar–agar (AA). Film thickness and density increased with increase in concentration of hydrocolloids and the respective highest value 0.199 mm and 2.02 g/cm3 were found for the film possessing 2% AA. The film barrier properties varied with hydrocolloids and the lowest value of water vapour transmission rate (1494.54 g/m2) and oxygen transmission rate (0.020 cm3/m2) was observed for the film with 1% XG and 1.5% AA, respectively. Mechanical and thermal properties also improved upon addition of hydrocolloid. Highest tensile strength (20.14 MPa) and glass transition temperature (150.6 °C) was observed for film containing 2% AA. Fourier transform infrared spectroscopy demonstrated the presence of –OH, C–H, and C=O groups. The change in crystallinity was observed through peak in X-ray diffraction analysis, which increased with increase in the hydrocolloids’ concentration.
Similar content being viewed by others
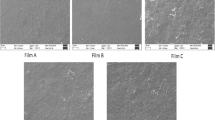
Explore related subjects
Discover the latest articles, news and stories from top researchers in related subjects.Avoid common mistakes on your manuscript.
Introduction
Nowadays, biodegradable and edible packaging materials have gained enormous attention and the industry is experiencing a paradigm shift from plastic to biodegradable-edible packaging material. Biodegradable-edible films are generally made up of biodegradable polymers in the combination of hydrocolloids. Different natural biopolymers such as starch, cellulose, fibre, pectins, gums, gluten, zein waxes, fatty acids, polylactic acid, pullulan, polyhydroxyalkanoates etc. are commonly used to give compostability attributes to edible film. These components are better to incorporate as they leave no toxic residues. Among the different biopolymers, starch has been identified as a potential source for the development of edible films owing to their wide availability in nature and low cost (Saberi et al. 2017; Nawab et al. 2017). However, the inherent properties of starch (insoluble nature, retrogradation etc.) and of the final derived or developed films (poor mechanical strength, brittleness, high moisture sensitivity and inadequate barrier properties) restrict the use of starch in pure form for intended purpose. This favors the need of blending starch with other chemicals and hydrocolloids and achieves better results while overcoming above mentioned limitations (Saberi et al. 2017). Several studies were reported about the preparation of edible films using blends of starches and hydrocolloids like guar gum and pea starch (Saberi et al. 2017), potato starch and gelatin (Podshivalov et al. 2017), sago starch and fish gelatin (Al-Hassan and Norziah 2012), corn starch and gelatin (Zhang et al. 2013) etc., and acknowledged different observations like improved transparency and toughness, water solubility, film thickness, tensile strength and water–vapor barrier property. It may thus be stated that the properties of edible film are not only dependent on the characteristics of starch i.e. amylose content, amylose–amylopectin ratio, linearity of amylose chains and degree of branching, but also depends on the interaction behavior of starch and hydrocolloids.
Elephant foot yam (EFY) is one of the natural and rich sources of starch. Literature review demonstrated limited utilization of EFY starch for development of edible or biodegradable films. Among the different reported studies, it was found that pure EFY starch based films are sticky and difficult to handle (Sukhija et al. 2018). Hence, attempts were made to develop yam starch based biodegradable-edible film in the combination with glycerol, sucrose, whey protein, propylene oxide, sodium trimetaphosphate and sodium tripolyphosphate (Wang et al. 2017; Mali et al. 2002; Rugchati and Thanacharoenchanaphas 2015). These studies were mainly focused on the effect of concentration (starch and hydrocolloid) on film forming abilities and quality attributes of developed film. Mali et al. (2005) reported important effect of glycerol on mechanical properties of yam based films. Increase in glycerol concentration resulted in puncture deformation, enhanced strain at break, decreased young modulus and puncture force. The study concluded that yam based films may possess tensile strength equivalent to low-density poly ethylene films but often accompanied with lower deformation. Rugchati and Thanacharoenchanaphas (2015) also reported prominent effect of glycerol and starch concentration on tensile properties of developed film. Also, it was found that the water vapor permeability and oxygen transmission was critical to the glycerol concentration. The study also moulded the film in form of bag, exhibit the probability of its commercialization. Wang et al. (2017) explored the effect of propylene oxide, sodium trimetaphosphate and sodium tripolyphosphate on the film forming property of yam starch. It was found that these treatments or chemicals affected the transparency, tensile strength and elongation at break properties of film. The viscosity of few solutions prepared after combinations of starch and chemicals were found non-suitable for development of film. The starch film modified with 8% propylene oxide, sodium trimetaphosphate and sodium tripolyphosphate exhibited best tensile strength.
Despite of these advancements reported on yam starch based edible films, it was observed through literature review that very few to almost none of the studies had utilized EFYS and hydrocolloids (XG/AA) for the development of edible film. Hence, the present study was aimed at investigating the interaction effect of hydrocolloids (XG and AA) and EFYS on properties of developed edible films and to evaluate the potential of the developed film as a packaging material.
Materials and methods
Materials
Elephant foot yam was procured from local market in Kundli, Haryana, India and the starch was extracted by the method described by Singh et al. (2005). The detail composition of EFYS is described by Nagar et al. (2019). Xanthan gum (Molychem, India), agar–agar (Merck, India) and glycerol (SRL, India) of analytical grade were used for the development of edible film.
Film preparation
EFYS based edible films were prepared by the method described by Saberi et al. (2015). The filmogenic solution was prepared by dispersing 4 g EFYS and hydrocolloids (AA and XG) at 0.5 g, 1 g, 1.5 g and 2 g concentration levels in 100 mL of distilled water. Suspensions were gently heated with continuous stirring. Furthermore, 40% (w/w) glycerol was added to the film suspension as a plasticizer and heated at 95 °C (30 min) for complete gelatinization of starch. The gelatinized film suspensions were cooled to room temperature with gentle stirring to remove the air bubbles. The 30 mL film suspension was poured in petri-plates (9 cm dia.) and dried at 45 °C for 12 h. Films were peeled off carefully from the petri-plates and conditioned in a desiccator at 60 ± 5% relative humidity for 24 h prior to further testing.
Moisture content measurement
Film moisture content was determined using the hot air oven method, where film was (dried at 105 °C for 24 h) (Khazaei et al. 2014). The difference in the weight of the film before and after drying was measured, and the moisture content (M. C.) in wet basis (w.b) of the film was calculated using Eq. 1.
Thickness and density measurement
The thickness of films was measured by using digital micrometer (least count: 0.001 mm, Mitutoyo). For each film, thickness measurement was taken at five random places at different regions and average value was calculated. Film density was calculated by measuring the mass and volume. The volume of the film was calculated by multiplying the thickness with sample surface area (Saberi et al. 2016).
Color properties
The color of the films was measured by using hand hold Chroma-meter (KONICA MINOLTA, CR-400, Japan). Background measurement was performed using standard white plate. Samples were placed in plastic container and the chromatic values a* (greenness to redness), b* (blueness to yellowness) and L* (lightness) were measured in triplets (Nandi and Guha 2018). Total color difference was calculated (Eq. 2) with respect to control sample by having mean of three value of \({\text{L}}_{\text{c}}^{*} ,{\text{a}}_{\text{c}}^{*} \,\,{\text{and}}\,\,{\text{b}}_{\text{c}}^{*}\) chromatic values.
Optical properties
The transparency of films was evaluated by using UV-spectrophotometer (Model no.UV-2600 240 V EN, Japan). The films were cut into small rectangular pieces and placed in the spectrophometric cell. The light intensity was measured at 560 nm at 25 ± 2 °C. Analyses were performed in duplicate and the transparency was determined using the following equation.
where A is the absorption of film at 560 nm with the specimen with respect to no specimen as blank (Maran et al. 2013).
Thermal properties
Thermal properties of films were evaluated using Differential Scanning calorimeter (DSC. NETZSCH, Germany). The films were cut into small pieces (10 ± 0.05 mg) and hermetically sealed in aluminum crucible pan, and kept for 1 h at room temperature. The hermetically sealed pans were heated from 20 to 200 °C (10 °C/min) with empty aluminum pan as reference. The onset temperature (To), peak temperature (Tm), end temperature (Te), were calculated from the thermograms (Tongdeesoontorn et al. 2011).
Water vapor transmission rate (WVTR)
WVTR of films was estimated gravimetrically using modified ASTM (1996) method using Lab think water vapor transmission rate tester (PERME W3/030). The test cups were 2/3rd filled with distilled water to create 100% relative humidity inside the wet chamber. The film samples were cut into specimen area 33 cm2, sealed over the circular opening of the cup and then placed in a test dish installation chamber at 75% RH and 25 °C. Water vapor permeability was determined from the weight gain of the test cup after every 30 min over a period of 12 h. The WVTR and coefficient of permeance were calculated using W3010 (CH&EN) 1.12.020 version software.
Oxygen transmission rate (OTR)
The oxygen transmission rate (OTR) of films was measured according to ASTM (2004) with some modification by Lab think (PERME 0X2/230) oxygen transmission rate analyzer. EFYS films were masked with aluminum foil with the exposed test area of 50 cm2. The film samples were placed into test cell having oxygen (concentration 21%) in one side and carrier gas nitrogen (concentration 98%) at other side, with 101 kPa pressure maintained in both the chamber. All the test samples were analyzed at 25 °C and 50% RH. The measurements were run for 2 cycles (60 min) for each sample for 12 h.
Functional group analysis of film
Functional group analysis of films was performed using FTIR spectrometer (ALPHA Bruker, Germany) at room temperature. Attenuated total reflection (ATR) plate was cleaned using isopropyl alcohol and scanning was performed over the range of 4000–400 cm−1 taking 24 scans for the samples. The background spectrum was scanned under the same parameter before each sample scanning (Xu et al. 2005).
XRD analysis
X-ray diffraction (XRD) analysis of films was performed using Ultimate IV X-Ray Diffractometer (Rigaku) at 40 kV and 40 mA with K-beta filter. Film samples were conditioned in desiccator at 58% RH and scanned in the range of (2θ) 5–80° at rate 8°/min. The percentage relative crystallinity was calculated according to procedure described by Nara and Komiya (1983). Firstly, crystalline and amorphous region was separated by a smooth curve connecting each points of minimum intensity and then a straight baseline was drawn joining the two points of minimum intensity at 2θ of 5 and 50°. The upper region of smooth curve was crystalline region while region between smooth curve and baseline was amorphous region. The ratio of crystalline region to overall area was calculated as relative crystallinity.
Texture analysis
The mechanical properties of the films were determined using a TA-XT texture analyzer (Stable Micro Systems, UK). The films were conditioned for 48 h at 55% RH at room temperature in desiccator. The film strips of 5 cm × 1 cm were fixed in grip with initial distance of 30 mm and crosshead speed maintained at 0.83 mm/sec. Tensile strength (TS, MPa), maximum elongation percentage at break (EAB, %) and Young’s modulus (YM, MPa) was calculated using Eqs. 4, 5 and 6 respectively
where Fmax is the maximum force, A is cross sectional area of film, Lmax and L0 are maximum deformation before breakage and initial length respectively, S is slope of force deformation curve (Nawab et al. 2017).
Statistical analysis
All the experiments were carried out in triplets but transparency and thermal analysis were performed in duplicates. Significance differences among means were evaluated using one-way ANOVA performed at a probability level of p < 0.05 in SPSS software (Version 25.0, SPSS Inc., Chicago, IL, USA). Homogeneous group were determined using Duncan test with p < 0.05.
Results and discussion
Moisture content, thickness and density of film
Physical properties such as moisture content, thickness and density of the prepared film samples are presented in Table 1. Moisture content of film help in understanding the interaction of EFYS with AA and XG, and will also affect the water binding capacity of the film. Moisture content of the EFYS film was found to be 23.66% (w.b.), which increased with the increase in concentration of AA (26.42% at 2%) and XG (24.36% at 2%). Addition of hydrocolloids resulted in higher hydrogen bonding with water whereas higher concentration of hydrocolloid restricted the penetration and evaporation of water from starch granules due to wrapping of starch granules. Presence of hydrocolloids improved the hydrophilic nature of starch molecules resulting in higher water absorption, consequently higher molecule entanglement and increase in viscosity favoring higher moisture retention during its’ drying (Singh et al. 2015). Haziraha et al. (2016) have found increase in moisture content (21.97–23.61%) of gelatin-carboxymethyl cellulose film blends XG (0.2–1%).The effect of AAG on moisture content is also supported by data of Maran et al. (2013) for edible film from tapioca starch and AAG.
Thickness and density are very important parameters to control the mechanical and barrier properties of the film and are capable to bring change in the structure of film during drying. The thickness and density of the film significantly increased with increase in the concentration of XG (Thickness 0.163 mm to 0.187 and density 1.73 ± 0.01 to 2.02 ± 0.00 g/cm3) and AA (Thickness 0.163 to 0.199 mm and density 1.73 ± 0.01 to1.98 ± 0.00 g/cm3) concentration. Increase in thickness might be due to the difference in the concentration of film solutions where the dry matter content increased upon addition of hydrocolloids with respect to constant volume. The resulted increase in dry matter further increased the density of film. In addition to that, the increase in density may also be attributed to strong inter-molecular H-bonding between molecules resulting in compact structure on addition of hydrocolloids (Saberi et al. 2017). The trends observed for density in the present study is supported by Saberi et al. (2017) for density of pea starch and guar gum based film. They reported that the increase in amount of guar gum resulted in increase in dry weight of the film and develop strong hydrogen bonding between starch and gum molecules which increased the thickness. Phan et al. (2009) also reported that the addition of arabinoxylan on cassava starch increases the thickness and density of cassava films due to the change in dry matter concentration and through retractibility of film during drying owing to evaporation of water and strong compact structure of the film. Additionally, the difference in molecular weight, composition, interaction components and development of hydrogen bonding positively affected the thickness and density of film.
Color properties
Film color values L*, a* and b* are represented in Table 1. L*, a* and b* values of EFYS film was found to be 35.12, 0.36 and − 2.49, respectively. Addition of XG and AA did not affect the chromatic color values significantly (p < 0.5). The color value L* and b* increased from 35.12 to 39.41 and − 2.49 to − 1.93 respectively whereas a* value decreased from 0.36 to − 0.11. Total color difference (∆E) decreased non-significantly from 5.27 to 5.10 for AAG but increase from 5.24 to 5.81 for XG with increase in concentration of hydrocolloids and this might be due to the increase in L* and b* value, resulting in an increase in lightness and yellowness of the film, respectively. The variation in the chromatic values with increase in concentration of hydrocolloids might also be due to the change in surface light absorption with increase in dry solid. The higher concentration of hydrocolloids resulted in slightly reddish blue color close to neutral gray. Similar findings were also reported by Nawab et al. (2017) for mango kernel starch based film with increase in concentration of xanthan and guar gum from 10 to 30%.
Transparency
Transparency (%) measures the amount of light passed through the material (Saurabh et al. 2015). Transparency of EFYS film (control) was 20.27%. Addition of AA (0.5–1.5%) increased the transparency (25.40–33.57%) whereas reduction (31.40–20.04%) was observed for XG (0.5–2%). The highest transparency of film was observed for 1.5% concentration of AA which results in lower opacity. The reduction in transparency on addition of hydrocolloids probably is due to the interaction of amylose and amylopectin molecules responsible for the formation of compact and higher polymeric chain network restricting the passage of light and modifying the refractive index (Ortega-Toro et al. 2014). The increase in transparency with AA might be due to less wrapping of starch molecules during interaction between starch and AA molecules. The overall transparency of EFYS based films were very less which blocked the incidence of visual light and can be effectively utilized to prevent the photon based oxidation. Haziraha et al. (2016) also reported the similar findings for composite gelatin CMC-xanthan films.
Oxygen transmission rate (OTR) and oxygen permeability (OP)
Many degradation reactions like oxidation, rancidity and enzymatic browning occurs in food and accelerated in the presence of oxygen. Hence, the oxygen transmission through the packaging film is an important parameter to estimate the shelf life, sensory properties and nutrition value of wrapped food products. Thus, lower oxygen permeability of biopolymer films over synthetic films protects the food from deteriorative effect of oxygen. EFYS film has maximum (0.053 cm3/m2.24 h) OTR and it get reduced as the hydrocolloid concentration increased compared to control. OTR varied in the range of 0.053–0.017 cm3/m2.24 h and 0.053–0.026 cm3/m2 24 h with the change in concentration of for AA and XG (Table 2). Similar to OTR, reduction in OP was observed from 1.063 × 10−14 g cm/cm2 s Pa (EFYS film) to 5.39 × 10−15g cm/cm2.s.Pa and 5.48 × 10−15 g cm/cm2 s Pa at 1.5% AA and 2% XG respectively, and showed the better resistant to OP as compared to other film samples. This might be due to the intermolecular hydrogen bonding between the starch and hydrocolloids which led to formation of compact structure of polysaccharides limiting intermolecular chain mobility and reduces the free space between starch molecules (Shen et al. 2010). Also, increase in crystallinity of film makes film impermeable and hence, lower the OP (Nawab et al. 2017).Contrarily, adding XG up to 1.5% didn’t affect the OP satisfactorily. It is normally accepted that addition of hydrocolloid contributes to the decrease of OP, however a higher concentration of gum is required to increases OP (Caner et al. 1998). Results were supported by Shen et al. (2010) for chitosan based potato starch based film.
Water vapor permeability (WVP)and Water vapor transmission rate (WVTR)
WVP of packaging film is a quality parameter directly affecting the quality of packed food. Partial vapor pressure difference across the packaging film is a trigger force for moisture diffusion. However, characteristics of base material such as presence of polar groups and polysaccharides will also influence the permeability. WVP for EFYS film was 1.91 × 10−11 g cm/cm2 s Pa and overall reduction was observed with increase in concentration of AA and XG (Table 2). The WVP of EFYS/AA/XG film was found in the range of 10−11–10−12 g cm/cm2 s Pa, which was in the range of result reported by Nawab et al.(2017) for the composite film of mango kernel starch and guar gum (2.96 × 10−10 to 4.29 × 10−10 g m/m2 s Pa) and Arismendi, et al. (2013) for xanthan gum/tapioca starch edible film (2 × 10−9 g/m s Pa). Similarly, Mali et al. (2002) also reported the WVP in the range of 0.96 × 10−10–1.81 × 10−10g m/m2 s Pa for the yam starch based film. Lower concentration of hydrocolloid increased the WVP, however the higher concentration led to reduction in WVP. Hydrocolloids are hydrophilic in nature and at lower concentration facilitate the water molecules to make bond with active side of polymer chain and thus, created micro cavities. Hence, the mobility of the polymer chain increased which increased the diffusion rate (Nandi and Guha 2018). Starch-based films with high WVP can be used in modified atmospheric packaging of fruits and vegetable where higher moisture diffusion is required (Guilbert 2000). At higher concentration, intermolecular hydrogen bonds between hydrocolloids and starch molecules could possibly reduce the free –OH groups that can react with water vapor molecules. This, further increase the viscosity during gelatinization and form cohesive film with good barrier properties (Dang and Yoksan 2015). During morphological characterization (Nagar et al. 2019), it was found that both the hydrocolloids partially wrapped and adhered to the surface of starch granules, which reduced the starch’s free hydroxyl group. The reduction in free hydroxyl group results in less reaction with water molecules which reduce the channel formation and consequently led to the reduction of WVP.
The WVTR of composite film ranged from 1783.64 to 505.46 and 2722.42 to 1663.64 g/m2 24 h for EFYS-AA and EFYS-XG film, respectively. WVTR was higher (2386.67 g/m2 24 h) for EFYS film and decreased with increase in the concentration of AA and XG. The film prepared at 1% XG had better barrier property with minimum WVTR (1494.54 g/m2 24 h). Reduction in WVTR with increase in the concentration may be due to increase in dissolved solid mass which results in denser solid matrix structure with high solid concentration per unit area (Rompothi et al. 2017). Another reason could be that the addition of hydrocolloids results in strong inter and intra molecular H-bonding between starch and hydrocolloids molecules thereby increasing the apparent viscosity during gelatinization and forms cohesive film with good barrier properties.
Thermal characteristics
Thermographs of EFYS film along with hydrocolloid blends (Table 3) were found to be consisting of a single endothermic sharp peak, possibly due to the homogeneity of films (Tongdeesoontorn et al. 2011).The endothermic peak was associated with the melting of crystalline molecules of starch during the retrogradation (Almasi et al. 2010). The endothermic peak of melting consists of onset, mid (melting) and end temperature. The melting temperature decreased at lower concentration (0.5%) of hydrocolloid compared to control but increased with further increase in concentration of hydrocolloid. The melting temperature (Tm) of EFYS film was 121.8 °C and the highest melting temperature i.e. 150.6 °C and 137.8 °C was observed for the film having 2% concentration of AA and XG respectively. The variation in melting temperature might be due to the interaction of EFYS and AA/XG, which interrupted the arrangements of chains of starch polymer and reduced the glass transition temperature at lower concentration. However, the higher crystallinity imparts high melting temperature at higher concentration of hydrocolloids. Tongdeesoontorn et al. (2011) also reported low melting temperature of cassava starch film blended with CMC compared to cassava starch film.
FTIR analysis of film
The interaction between EFYS and hydrocolloids EFYS based edible films was observed using FTIR spectrophotometer. Four main peaks were observed at 3269 cm−1, 2927 cm−1, 1343 cm−1 and 997 cm−1, corresponding to X–H broad band region, double bond region and finger print region respectively (Fig. 1). The stretch broad band occurring at 3269 cm−1 was associated with free intermolecular –OH group present in the starch granules and also to the absorption of water during the gelatinization of the starch–hydrocolloids mixture used for film preparation. The sharp peak observed at 2927 cm−1 was associated with C–H stretching group. The stretched band for alkanes was observed between 3000 and 2840 cm−1. The lower peak observed at 1646 cm−1 confirmed the presence of water molecules incorporated during starch gelatinization. Furthermore, weak and broad band was observed for C–O–H group at 1343 cm−1. Pavia et al. (2009) reported 1440 cm−1 to 1220 cm−1 as the band shift for C–O–H group due to intra molecular hydrogen bonding in the molecules. Also, in the present study, variation in the structure resulted in the strong and sharp band at 998 cm−1 under finger print region ranging from 1200 to 950 cm−1.
The addition of XG and AA had shown the same characteristics in FTIR spectra with respect to various chemical compounds but exhibited differences in peak absorbance and intensity of broad band. The peak absorbance was observed to be higher for both XG and AA at 0.5% and 1% concentration level, whereas peak absorbance for EFYS control and higher concentration levels of AA and XG was found lower. The shift in absorbance of –C=O stretching bonds upon addition of hydrocolloids was due to rupturing of intermolecular hydrogen bonding between carboxyl group. Formation of ester bond also occurred due to interaction between –OH molecules of amylopectin and –COOH molecules of hydrocolloids, and was also reported for chitosan based edible film (Xu et al. 2005). Results showed that addition of hydrocolloids at lower concentration level to the starch formed more stable structure and increased the stability compared to EFYS control film, whereas hydrocolloids at higher concentration level resulted in the high interactions and enhanced association between starch and hydrocolloids compared to water.
XRD analysis
The XRD analysis of EFYS based edible film was carried out to study the crystalline and amorphous behavior of prepared film (Table 3 and Fig. 2). EFYS film consists of a hump between 2θ of 17° and 22° which describe amorphous behavior of film (Angellier et al. 2006). Addition of AA and XG has different effect on the crystallinity of film as compared to EFYS film. However, addition of hydrocolloids did not change the diffraction pattern of films. The percentage crystallinity of EFYS film was found to be 36.02%, which was increased after addition of hydrocolloids at low concentration (0.5%). Further increase in concentration from 0.5 to 2%, reduced the percentage crystallinity of XG films from 39.06 to 38.12% and increased in AA films from 36.63 to 42.79%. Findings were also supported by Nawab et al. (2017) and Wang et al. (2018) for the mango kernel starch and Nano-bacterial cellulose based film respectively. They also reported reduction in crystallinity in mango kernel starch and XG based edible film without change in the diffraction pattern, along with improvement in crystallinity of nano-bacterial cellulose and agar based edible film respectively. The reduction in crystallinity of XG films might be due to hindrance effect of gum which limits the ordering and arrangement during cooling and retrogradation of starch molecules. The crystallinity of starch based film is associated with the retrogradation of starch molecules after gelatinization. The crystalline region consist of ordered arrangement of molecules due to parallel and closely packed chains, whereas the molecular chains are disordered and without structure in the amorphous region (Robertson 2013). Amylose and amylopectin are two main components of starch and form long and highly branched chain respectively, responsible for the low crystallinity of starch based film (Robertson 2013).
Textural analysis
A sufficient mechanical strength of packaging film is required to resist external stress and maintain its integrity. Table 4 shows the effect of different concentration of XG and AA on the mechanical properties of EFYS film. The results suggest that addition of hydrocolloids in starch produced a reinforcing effect on tensile strength (TS) which significantly (p < 0.05) improved from 15.81 MPa (EFYS film) to 17.30–20.14 MPa and 19.27–19.48 MPa with the addition of AA and XG, respectively. These trends are in line with mango kernel starch-gum composite films (Nawab et al. 2017) and tapioca starch/xanthan gum composite films (Arismendi et al. 2013). The improvement in TS may be due to the development of intermolecular H-bonds and the cohesive molecular structure of the films after addition of hydrocolloids (AA/XG). However, the negative trend for maximum elongation percentage at break (EAB) was observed with the addition of hydrocolloid. EFYS film had maximum EAB (23.96%), which reduced to 19.75–13.34% and 21.52–14.69% with the addition of AA and XG from 0.5 to 2%, respectively. Similar findings were reported by Nawab et al. (2017) and Arismendi et al. (2013) for the mango kernel starch-gum composite films and tapioca starch-xanthan gum composite films, respectively. The reduction in EAB could be explained by a synergistic interaction between the EFYS and hydrocolloid molecules that produces a more unfolded network due to weaker amylose–amylose interaction forces (Saberi et al. 2017).
The Young Modulus (YM) signifies the stiffness of the film and was significantly (p < 0.05) varied with the addition of hydrocolloids. The YM of EFYS film was 54.08 MPa and increased in the range of 60.81–65.08 MPa and 56.15–69.77 MPa with the increase in concentration of AA (0.5–1.5%) and XG (0.5–2%), respectively. The increase in YM with the addition of hydrocolloid may be due to increase in cohesiveness which offers greater resistance against the deformation. In addition to that, the enhanced H- bonding, ordering polymer alignment and improve structural orientation during retrogradation also contributed to YM. Results were also supported by Nawab et al. (2017) for mango kernel starch-gum composite films.
Conclusion
Elephant foot yam starch based edible film was developed. Moisture content, thickness and density of the EFYS film increased with the increase in hydrocolloids. Film exhibited better interaction with AA compared to XG, in terms of mechanical and thermal properties. The highest mechanical strength (20.14 MPa) and glass transition temperature (150.6 °C) was observed for film having highest percent of agar–agar (i.e. 2%). Good mechanical properties of film may prevent the stored products from mechanical stress. and also explores its possibility for the replacement of plastic packages. Film made from 1.5% agar–agar possessed lowest barrier properties (oxygen permeability), making it apt for storing fruits and vegetables which require higher transmission rate.
References
Al-Hassan AA, Norziah MH (2012) Starch-gelatin edible films: water vapour permeability and mechanical properties as affected by plasticizers. Food Hydrocolloids 26(1):108–117
Almasi H, Ghanbarzadeh B, Entezami AA (2010) Physicochemical properties of starch-CMC-nanoclay biodegradable films. J Biol Macromol 46:1–5
Angellier H, Molina-Boisseau S, Dole P, Dufresne A (2006) Thermoplastic Starch–Waxy maize starch nanocrystals nanocomposites. Biomacromolecules 7(2):531–539. https://doi.org/10.1021/bm050797s
Arismendi C, Chillo S, Conte A, Del Nobile MA, Flores S, Gerschenson LN (2013) Optimization of physical properties of xanthan gum/tapioca starch edible matrices containing potassium sorbate and evaluation of its antimicrobial effectiveness. LWT Food Sci Technol 53(1):290–296
ASTM (1996) Standard test methods for water vapor transmission of materials-method E-96. In: Annual book of ASTM. American Society for Testing and Materials, Philadelphia
ASTM (2004) Standard test method for oxygen gas transmission rate through plastic film and sheeting using a coulometric sensor, method D 3985–05. American Society for Testing and Materials, Philadelphia
Caner C, Vergano PJ, Wiles JL (1998) Chitosan film mechanical and permeation properties as affected by acid, plasticizer, and storage. J Food Sci 63(6):1049–1053
Dang KM, Yoksan R (2015) Development of thermoplastic starch blown film by incorporating plasticized chitosan. Carbohydr Polym 115:575–581
Guilbert S (2000) Edible films and coatings and biodegradable packaging. Bull Int Dairy Fed 346:10–16
Haziraha N, Isab MIN, Sarbon NM (2016) Effect of xanthan gum on the physical and mechanical properties of gelatin-carboxymethyl cellulose film blends. Food Packag Shelf Life 9:55–63
Khazaei N, Esmaiili M, Djomeh ZE, Ghasemlou M, Jouki M (2014) Characterization of new biodegradable edible film made from basil seed (Ocimum basilicum) gum. Carbohydr Polym 102:199–206
Mali S, Grossmann MVE, Garcia MA, Martino MN, Zaritzky NE (2002) Microstructural characterization of yam starch films. Carbohydr Polym 50(4):379–386
Mali S, Grossmann MVE, Garcia MA, Martino MN, Zaritzky NE (2005) Mechanical and thermal properties of yam starch films. Food Hydrocolloids 19(1):157–164
Maran JP, Sivakumar V, Thirugnanasambandham K, Sridhar R (2013) Response surface modeling and analysis of barrier and optical properties of maize starch edible films. Int J Biol Macromol 60:412–421
Nagar M, Sharanagat VS, Kumar Y, Singh L, Mani S (2019) Influence of xanthan and agar–agar on thermo-functional, morphological, pasting and rheological properties of elephant foot yam (Amorphophallus paeoniifolius) starch. Int J Biol Macromol 136:831–838
Nandi S, Guha P (2018) Modelling the effect of guar gum on physical, optical, barrier and mechanical properties of potato starch based composite film. Carbohydr Polym 200:498–507
Nara S, Komiya T (1983) Studies on the relationship between water-satured state and crystallinity by the diffraction method for moistened potato starch. Starch Stärke 35(12):407–410
Nawab A, Alam F, Haq MA, Lutfi Z, Hasnain A (2017) Mango kernel starch-gum composite films: physical, mechanical and barrier properties. Int J Biol Macromol 98:869–876
Ortega-Toro R, Jimenez A, Talens P, Chiralt A (2014) Properties of starch hydroxyl propyl methylcellulose based films obtained by compression molding. Carbohydr Polym 109:155–165
Pavia DL, Lampman GM, Kriz GS (2009) Introduction to spectroscopy: a guide for students of organic chemistry, 4th edn. Harcourt College Publishers, Belmont, pp 15–104
Phan TD, Debeaufort F, Voilley A, Luu D (2009) Biopolymer interactions affect the functional properties of edible films based on agar, cassava starch and arabinoxylan blends. J Food Eng 90:548–558
Podshivalov A, Zakharova M, Glazacheva E, Uspenskaya M (2017) Gelatin/potato starch edible biocomposite films: correlation between morphology and physical properties. Carbohydr Polym 157:1162–1172
Robertson GL (2013) Food packaging: principles and practice, 3rd edn. CRC Press, Boca Raton, pp 11–90
Rompothi O, Pradipasena P, Tananuwong K, Somwangthanaroj A, Janjarasskul T (2017) Development of non-water soluble, ductile mung bean starch based edible film with oxygen barrier and heat sealability. Carbohydr Polym 157:748–756
Rugchati O, Thanacharoenchanaphas K (2015) Application of biodegradable film from yam (Dioscorea alata) starch in Thailand for agriculture activity. Int J Environ Rural Dev 6(1):28–33
Saberi B, Vuong Q, Chockchaisawasdee S, Golding JB, Scarletta CJ, Stathopoulosc CE (2015) Water sorption isotherm of pea starch edible films and prediction models. J Food Sci 5(1):1–6
Saberi B, Thakur R, Vuong QV, Chockchaisawasdee S, Golding JB, Scarletta CJ, Stathopoulosc CE (2016) Optimization of physical and optical properties of biodegradable edible films based on pea starch and guar gum. Ind Crops Prod 86:342–352
Saberi B, Thakur R, Bhuyan DJ, Vuong QV, Chockchaisawasdee S, Golding JB, Scarletta CJ, Stathopoulosc CE (2017) Development of edible blend films with good mechanical and barrier properties from pea starch and guar gum. Starch Starke 69:1–15
Saurabh CK, Gupta S, Bahadur J, Mazumder S, Variyar PS, Sharma A (2015) Mechanical and barrier properties of guar gum based nano-composite films. Carbohydr Polym 124:77–84
Shen XL, Wu JM, Chen Y, Zhao G (2010) Antimicrobial and physical properties of sweet potato starch films incorporated with potassium sorbate or chitosan. Food Hydrocolloids 24(4):285–290
Singh S, Raina CS, Bawa AS, Saxena DC (2005) Effect of heat-moisture treatment and acid modification on rheological, textural and differential scanning calorimeter characteristics of sweet potato starch. J Food Sci 70:374–378
Singh T, Chatli M, Sahoo J (2015) Development of chitosan based edible films: process optimization using response surface methodology. J Food Sci Technol 52:2530–2543
Sukhija S, Singh S, Riar CS (2018) Physical, mechanical, morphological, and barrier properties of elephant foot yam starch, whey protein concentrate and psyllium husk based composite biodegradable films. Polym Compos 39:407–415
Tongdeesoontorn W, Mauer LJ, Wongruong S, Sriburi P, Rachtanapun P (2011) Effect of carboxymethyl cellulose concentration on physical properties of biodegradable cassava starch-based films. Chem Cent J 5(6):1–5
Wang L, Liu X, Wang J (2017) Structural properties of chemically modified Chinese yam starches and their films. Int J Food Prop 20(6):1239–1250
Wang X, Guo C, Hao W, Ullah N, Chen L, Li Z, Feng X (2018) Development and characterization of agar-based edible films reinforced with nano-bacterial cellulose. International Journal of Biological Macromolecule 118(A):722–730
Xu YX, Kim KM, Hanna MA, Nag D (2005) Chitosan-starch composite film: preparation and characterization. Ind Crops Prod 21:185–192
Zhang N, Liu H, Yu L, Liu X, Zhang L, Chen L, Shanks R (2013) Developing gelatin–starch blends for use as capsule materials. Carbohydr Polym 92(1):455–461
Acknowledgements
One of the author Mr. Mohit Nagar is thankful to Ms. Ria Bhadra (M. Tech., FPEM), NIFTEM for her support.
Author information
Authors and Affiliations
Corresponding author
Additional information
Publisher's Note
Springer Nature remains neutral with regard to jurisdictional claims in published maps and institutional affiliations.
Rights and permissions
About this article
Cite this article
Nagar, M., Sharanagat, V.S., Kumar, Y. et al. Development and characterization of elephant foot yam starch–hydrocolloids based edible packaging film: physical, optical, thermal and barrier properties. J Food Sci Technol 57, 1331–1341 (2020). https://doi.org/10.1007/s13197-019-04167-w
Revised:
Accepted:
Published:
Issue Date:
DOI: https://doi.org/10.1007/s13197-019-04167-w