Abstract
Hydrogen peroxide (H2O2) is one of the most popular and widely used oxidants. Among the wide range of synthesis techniques used for the production of H2O2; the electrochemical method allows the use of gas diffusion electrodes to generate H2O2 without limiting the low solubility of O2 in water. The present work reports the modification of carbon black with quinone, where the generation of H2O2 occurs in an electrochemical/chemical mechanism. The results obtained by this technique were found to be highly promising. The use of the organic compound 1,2-dihydroxyanthraquinone to modify carbon black electrode resulted in greater production of H2O2. Carbon black electrode modified with 1% of 1,2-dihydroxyanthraquinone yielded 298 mg L−1 of H2O2 at the end of 90 min of experiment, reaching an electrical efficiency of approximately 25.5%. Based on the findings of this study, H2O2 generation is found to be directly associated with the chemical structure of the carbon modifier and not solely related to the presence of quinone groups.

Graphical Abstract
Similar content being viewed by others
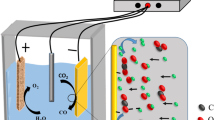
Explore related subjects
Discover the latest articles, news and stories from top researchers in related subjects.Avoid common mistakes on your manuscript.
Introduction
Hydrogen peroxide is normally produced by the reduction of O2 through quinone/hydroquinone equilibrium [1]. In this procedure, the catalytic reduction of 2-alkylanthraquinone in H2 yields anthrahydroquinone, the autoxidation of which promotes the formation of H2O2 [2,3,4,5]. The process requires the use of considerable amount of H2, and the highly active peroxide is extracted from organic medium and, subsequently, purified, concentrated, and delivered to the end user [6,7,8,9]. However, since peroxide is largely used for oxidizing organic pollutants, an alternative strategy would involve an on-site electrogeneration of H2O2, at a rate appropriate to the requirement for effluent treatment, through the reduction of O2 in dilute acid solution under mild conditions of current density and pH [4].
Perry (2019) has shed light on the wide range of methods employed for the synthesis of H2O2 [9]. The electrochemical technique can be performed using different types of electrodes; these include flat [10], reticulated [11,12,13], or porous electrodes [2, 14]. One will note, however, that carbon is the reference material when it comes to the reduction of O2 and formation of H2O2. Although the use of carbon as a support medium for O2 reduction reaction offers good selectivity for H2O2 generation, its underlying shortcoming lies in the low solubility of O2 in aqueous medium, especially in the processes in which the gas must be saturated in aqueous solutions.
The key advantage of the use of gas diffusion electrode (GDE) is that it helps to overcome the problems associated with the solubility of oxygen in aqueous medium. Through the application of this electrode, oxygen is supplied via the porous and hydrophobic structure of the GDE, allowing the reduction of O2 to occur without the limitation of mass transport [2, 14]. The porous structure provides a relatively large electrode area, which facilitates the reaction process [15,16,17,18].
The reduction of O2 in carbon involves a strong interaction of O2 with oxidized functional groups present on the carbon surface [19]. Among these functional groups, quinone compounds are specifically known to be directly involved in O2 reduction [20]. The ability of unmodified carbon black to generate H2O2 can be attributed to two characteristics; i carbon black is found to possess groups with oxygen on their surface; this facilitates the interaction of oxygen [4, 21]; and ii mobility of hydrogen in the carbonic structure. As noted by Radovic (2018), hydrogen presents mobility in graphite/carbon-based materials; as such, the movement of hydrogen is easier in systems with quinone/hydroquinone groups compared to polyaromatic molecular systems which do not contain these groups [20].
Considering that unmodified carbon has already been shown to produce H2O2 from the electrochemical reduction of oxygen [2, 4, 15, 16, 18, 21] coupled with the fact that hydrogen mobility is much easier in quinone/hydroquinone systems, the use of anthraquinones in carbon modification contributes to the enhancement of hydrogen mobility, thus leading to an increase in H2O2 generation.
Quinone compounds (Qo) have been shown to be efficient when it comes to H2O2 production beyond the confines of the traditional manufacturing process [2, 11, 12]. The following observations are made based on the equations below. In the reduction of anthraquinone, the uptake of a single electron produces the anthraquinone radical intermediate (Q●-) based on Eq. 1; while the acceptance of a second electron leads to the formation of an anthrahydroquinone (Qr), according to Eq. 2. The subsequent oxidation of anthrahydroquinone releases the electrons required for O2 reduction, based on Eqs. 3 and 4 [1, 2]:
This two-step reduction of quinone in solution generally leads to the formation of Q●- intermediate. The Q●- intermediate is found to have a short half-life, is relatively reactive, and can participate in O2 reduction reaction. In previously reported studies involving the use of compounds with quinone groups, the overpotential for O2 reduction on GDE made of carbon black matrix/PTFE modified with 10% (w/w) 2-ethylanthraquinone or 2-tert-butylanthraquinone was found to be dislocated by 400 mV toward less negative potentials [2, 12]. Furthermore, H2O2 generation was found to increase by 30% in the presence of the modifier, and the energy consumption for the generation of 1 kg of H2O2 was reduced to 232 kWh/kg−1 (a reduction of 60%).
The aim of the present work was to determine the electroactivity of Alizarin Red S (ALZ; 1,2-dihydroxyanthraquinone), menadione (MDA; 2-Methyl-1,4-naphthoquinone), and acenaphthoquinone (APQ; acenaphthylene-1,2-dione) used as modifiers for O2 reduction in acid medium. Carbon black/PTFE/modifier O2-fed cathodes were prepared with different amounts of carbon and organic modifiers, and the generation of H2O2 was optimized with respect to cell potential and modifier concentration.
Experimental
Voltammetric Experiments for O2 Reduction
Voltammetric experiments on RRDE were carried out using RRDE (from Pine Instruments), electrode with glassy carbon disc (GC), and a Pt ring (coefficient of collection N = 0.37). The modifiers were prepared by suspending conductive carbon black pigment (Degussa Printex 6 L) containing 0%, 0.5%, 1.0%, 2.0%, and 3.0% (w/w) of each organic compound in ultrapure water, isopropanol (4:1, v/v). The micro-layer was prepared as described in the literature [2, 14, 22].
All the materials investigated in this study were characterized electrochemically by cyclic voltammetry (CV) and linear sweep voltammetry (LV). The electrolyte was bubbled with N2 for 20 min before the first set of CV and LV measurements was performed. Thereafter, the electrolyte was bubbled with O2 for 40 min, and LV was performed again, while the O2 flow was maintained. Electrochemical measurements were performed at scan rates of 50 mV s−1 for CV and 5 mV s−1 for LV with electrode rotation in the range of 100–2500 rpm. All measurements were performed at room temperature in a three-compartment cell [2].
Controlled Potential Experiments with Gas Diffusion Electrodes
The precursor masses for the GDEs were prepared with carbon black (Degussa Printex 6 L), 60% dispersion of PTFE (DuPont Teflon® PTFE DISP 30) along with different amounts of each modifier (0%, 0.5%, 1.0%, 2.0%, and 3.0%). The GDE was constructed by the hot-pressing mechanism as described in the literature [2].
Controlled potential experiments were performed in a single compartment cell containing each of the GDEs, with exposed area of 19 cm2 as described previously [2], along with the same supporting aqueous electrolyte used in the voltammetric experiments. Electrolysis was carried out with applied current of 10, 20, 50, 75, and 100 mA cm−2 for 90 min (with sample collected every 15 min during the first 60 min and in 30 min intervals thereafter) and under 0.2 bar of O2.
The amount of H2O2 present in the electrolyte sample was determined by UV-Vis spectrophotometry using (NH4)6Mo7O24.4H2O (2.4 × 10−3 mol L−1) in H2SO4 (0.5 mol L−1) as described in the literature [2].
Voltammetric and controlled potential experiments were performed using an AutoLab-Metrohm PGSTAT-302 N with Pt as the counter-electrode and Ag/AgCl (KCl sat.) as the reference electrode. The supporting aqueous electrolyte (pH 1) contained H2SO4 (0.1 mol L−1) and K2SO4 (0.1 mol L−1).
Results and Discussion
Cyclic and Linear Voltammetric Experiments
In order to investigate the redox couples of the organic compounds, a microlayer of carbon black/modifiers was deposited on the GC disc of an RRDE, and cyclic voltammetric experiments were conducted in acidic sulfate solution saturated with N2 in the absence of O2 aiming at evaluating the reversibility of the chemical structure of the modifiers. Only the amount of 3% of each modifier was used; this is because at this percentage one can better observe the peaks of each material. The potential was scanned in the range of − 0.6 to + 0.6 V vs Ag/AgCl for ALZ and MDA, as well as in the range of − 0.6 to + 0.2 V vs Ag/AgCl for APQ. The i/E couples were recorded as shown in Fig. 1. With regard to APQ, the potential range of up to 0.2 V d was evaluated; this has to do with the high oxygen formation current in this potential range. At more positive potentials, the current reached higher peak currents compared to the peak related to oxidized form of the organic compound. The peaks observed were related to the oxidized structure (Qo) or reduced structure (Qr) of quinone groups in each of the modifiers [18,19,20,21], respectively; (Qo1) + 0,05 V/(Qr1) + 0,03 V for carbon black with 3.0% of ALZ, (Qo2) + 0,07 V/(Qr2) + 0,02 V for carbon black with 3.0% of MDA, and (Qo3) – 0.21 V/(Qr3) – 0,23 V for carbon black with 3% of APQ.
Voltammetric peaks at the potential described above are possibly related to the redox couples of – OH groups bound to the anthraquinone [2]. This organic compound structure is found to be very unstable in comparison with resonance-stabilized molecules. As a result, the current intensities associated with these peaks are smaller than those of the quinone/hydroquinone redox couples. It is likely that the peaks were detected in the present study due to the high concentration (3% w/w) of modifiers and the scan rate of 50 mV s−1 applied in the experiments.
Figure 2 shows the mechanism of reactions associated with the main redox pairs of modifiers adsorbed on the carbon surface, in the presence of O2, used for evaluating the H2O2 formation mechanism. In acid medium saturated with O2, the organic compound (structure [QO] in Fig. 2) is electrochemically reduced to the hydroquinone form [QR], while the latter is subsequently oxidized electrochemically to [QO]. However, with the saturation of O2 in the electrolyte, hydroquinone [QR] is oxidized chemically by the O2 present in solution to form [QO] along with the concomitant generation of H2O2, based on Eqs. 3 and 4 [4, 11, 18, 19]. To investigate the proposed scheme, the ORR process was studied by linear voltammetry in the potential range of + 0.4 to − 0.8 V vs Ag/AgCl. For each of the modifiers under investigation, a voltammogram was recorded for the electrolyte saturated with O2 discounting the current from the experiments with saturated N2.
The electrolyte solution was subsequently bubbled with O2, and the voltammogram was recorded again. The current associated with the ORR was obtained by subtracting the currents of the redox reactions, related to the electrolyte used (obtained by N2 saturation), from the total current (obtained by O2 saturation). In the present study, all the currents related to ORR were corrected by discounting the currents from the experiments with N2.
The linear voltammograms shown in Fig. 3 were obtained through the use of RRDE. The voltammograms indicate the catalytic activities of microporous layers of carbon black with different percentages of modifiers for the production of H2O2 from ORR. Plots of disc current (Id) as a function of applied potential exhibited similar behavior for all the modifiers investigated. For the unmodified carbon black, ORR began at a potential of around 0.0 V vs Ag/AgCl with the formation of limiting current at approximately − 0.6 V and maximum current of 480 μA at − 0.8 V. With regard to the materials containing 0.5% of modifiers, the reduction currents (Id) of the materials modified with ALZ and APQ presented currents of around 460 μA at − 0.7 V (close to those of the unmodified carbon), while the materials modified with MDA presented currents of around 510 μA at − 0.7 V (10% higher). With regard to other percentages of the modifiers employed, the limiting diffusion currents presented values similar to those obtained for the materials containing 0.5% of modifiers, with a variation of less than 5% between the currents. This behavior indicates that the addition of organic modifiers to the carbon does not exert any significant effect on ORR, regardless of the amount of modifiers used in the process.
Plots of ring current (Ir) as a function of applied potential are shown in Fig. 3. As described in the literature [22,23,24,25], when the Pt-ring is polarized at + 1.0 V vs Ag/AgCl, the current is associated with H2O2 oxidation; in this sense, the current observed in the ring is related to the amount of H2O2 detected on the electrode. In all the modifiers, the oxidation reaction of H2O2 in the ring began at a potential of approximately − 0.1 V, and the current limit, as determined by linear voltammetry, occurred at around 0.6 V. Considering all the modifiers investigated, carbon black with 1.0% ALZ presented the highest current of H2O2 detection. The APQ and MDA modifiers exhibited currents of H2O2 detection close to those of the unmodified carbon. In this sense, the addition of APQ and MDA did not favor the reaction of H2O2 formation from the electrochemical O2 reduction. It is worth noting that only ALZ (above 0.5% w/w) reached H2O2 detection currents higher than those of the unmodified carbon; the maximum current was observed for carbon modified with 1.0% of ALZ.
A comparison of the ring and disc currents showed that Id was close to 500 μA in all the modifiers and all the percentages investigated, while Ir for APQ and MDA presented currents close to those of the unmodified carbon. The modification of carbon with 0.5% ALZ yielded currents close to those of the unmodified carbon. Carbon modified with 1% of ALZ presented the highest current of H2O2 oxidation (160 μA at −0.8 V). With regard to carbon modified with the other two percentages of ALZ, a decrease was observed in the currents with the increase of the amount of ALZ; carbon modified with 3% ALZ presented the lowest current (135 μA at − 0.8 V) compared to the current recorded for unmodified carbon (123 μA at − 0.8 V).
This behavior is related to the parallel reactions that occur on the disc, which favor the ORR and, consequently, promote an increase in the production of H2O2. With regard to the case involving the use of ALZ, the amount of H2O2 on the disc and its detection on the ring may provoke interactions that exert influence over the analysis of RRO. One will note that the species are produced on a disc and they pass by the ring surface through the electrode rotation. Essentially, there is a direct relationship between the active areas of the ring and the disc as indicated by the coefficient of collection (N = 0.37) determined by the manufacturer of the electrode; the current detected on the ring is directly derived from the oxidation of H2O2 [26]. The efficiency of H2O2 generation (H2O2%) can be calculated according to Eq. 7 below [26]:
In applying this function, it is assumed that all of the currents on the ring are derived from the oxidation of H2O2. By doing so, H2O2% value of 100% would imply that the ORR was the only reaction on the disc and that its mechanism involved a 2-electron transfer resulting in the exclusive generation of H2O2. Based on the same parameters, the number of electrons (nt) involved in the oxidation reactions on the disc was calculated so as to gain an insight into the tendency of the ORR mechanism [27]:
Table 1 shows the values of H2O2% and nt for each of the catalysts investigated in this study compared with those of a commercial sample containing 20% Pt/C (E-TEK). The commercial sample is employed as a reference catalyst for ORR involving a 4-electron transfer mechanism [24]. The modification of carbon with 1% APQ and 1% MDA led to a minimum increase in the production of H2O2, with the maximum efficiency of 84% and 75%, respectively. With regard to the total number of electrons exchanged, the APQ and MDA modifiers did not show any differences in terms of H2O2 production, reaching the best values with 1% each of APQ and MDA (2.3 and 2.5, respectively). This behavior implies that the modification of carbon with APQ and MDA did not lead to an improvement in the generation of H2O2 in comparison with unmodified carbon.
Furthermore, the tendency of the ORR to follow a 2-electron mechanism, when carbon is modified with ALZ, shows that an increase in the amount of this organic compound led to an increase in H2O2 generation up to the percentage of 1% (of ALZ). The calculated values of nt refer to the number of electrons for all oxidation reactions that occur on the disc. One will note that the exact contribution of each mechanism to the ORR cannot be established through these nt values. Essentially, the nt values presented in Table 1 show that the addition of ALZ follows the pattern of 2-electron mechanism observed in the unmodified carbon electrode. This is different from the nt values obtained for Pt/C, which are related to a 4-electron mechanism.
The results shown in Fig. 3 and in Table 1 indicate that the modification of carbon electrode with APQ and MDA did not lead to improvements in H2O2 detection currents. By contrast, the modification of carbon electrode with ALZ promoted significant improvements in H2O2 detection currents. This analysis is based on the current values of the disc and the ring, where a comparison is made between the total reduction currents on the disc and the oxidation current on the ring. This comparative analysis appears to be insufficient to quantify the H2O2 generated in each of the materials investigated here. The analysis involved the construction of gas diffusion electrodes using the same materials employed in the RRDE studies.
Generation of H2O2 by Gas Diffusion Electrodes
Based on the data obtained from the voltammetric experiments, the use of carbon modifiers for the electrogeneration of H2O2 from ORR was found to be highly promising. In view of that, GDEs made of carbon black with and without ALZ were constructed, and the generation of H2O2 was analyzed quantitatively using spectrophotometric analysis. Electrolysis was carried out for 90 min under constant applied current in the range of 10–100 mA cm−2 using O2-fed GDEs which consisted of carbon black with 0, 0.5, 1.0, 2.0, and 3.0% of each modifier. It is worth pointing out that although the concentration of H2O2 present in the electrolyte was determined throughout the duration of the experiment, Fig. 4 shows the concentration at the end of each experiment for all the materials investigated.
Figure 4 shows the concentrations of H2O2 determined at 90 min using different current densities applied to GDEs modified with varying concentrations of the compounds investigated. In general, the MDA and APQ modifiers did not promote changes in H2O2 generation compared to unmodified carbon under all the percentages of modifiers evaluated. H2O2 generation reached a maximum of approximately 140 mg L−1 for both the carbon modified with MDA and APQ and the unmodified carbon.
The modification of carbon with ALZ promoted an increase in H2O2 generation compared to the results obtained for unmodified carbon. It is worth pointing out that no changes were observed in H2O2 generation when carbon was modified with 0.5% ALZ (Fig. 4a). The modification of carbon with ALZ at concentrations above 0.5% promoted an increase in catalytic activity, leading to an increase in H2O2 generation. Notably, when the carbon with 1% ALZ was used (Fig. 4b), an amount of 289 mg L−1 of H2O2 was generated; this is significantly higher than the amount of 134 mg L−1 of H2O2 generated when unmodified carbon was employed. Interestingly, when carbon was modified with ALZ concentrations higher than 1%, the increase in H2O2 generation was found to be lower than that observed for carbon modified with 1% ALZ. Precisely, the modification of carbon with 2% and 3% ALZ yielded 273 mg L−1 (Fig. 4c) and 210 mg L−1 (Fig. 4d) of H2O2, respectively.
Figure 4 shows the H2O2 concentration in each of the materials investigated. The experiments were performed in triplicate in order to determine the reproducibility of H2O2 generation and the stability of carbon modifiers at different applied current densities. By analyzing H2O2 concentration in the triplicate experiments, a maximum variation of 5.9 ± 0.6% was observed between the H2O2 concentrations in each condition. This variation range is very close to that reported by Reis (2012), who showed that gas diffusion electrodes have high reproducibility in the process of electrochemical H2O2 generation [28].
Another observation worth mentioning here regarding the variation of H2O2 concentration is the stability of carbon modifiers. The low variability in the triplicate experiments observed under the same conditions indicates the chemical stability of the modifiers. The same electrode used under five different current densities with three 90-min experiments each, totaling 22.5 h of experiments, presented a maximum variation of 5.9 ± 0.6% [28]. This shows that the modifier structure remained in equilibrium in both oxidized and reduced forms (Fig. 2), thus pointing to the chemical and electrochemical stability of the modifiers.
With regard to H2O2 generation, there has been a dramatic rise in the use of carbon modified with ALZ for O2 reduction. This can be attributed to an important feature of these modifiers: their ability to reduce O2 ceases in H2O2, in a process involving 2H+ and 2e−, as shown in the equations below:
where M(ads) represents ALZ, MDA or APQ.
Extended Mechanism
One of the key characteristics of quinones is their electrocatalytic profile in terms of H2O2 production. This behavior is linked to the addition of quinones to the structure of the modifiers investigated. This class of organic compounds (the quinones, to be precise) may exhibit typical characteristics of electron donors (mesomeric and inductive effects), as can be observed in Eq. 9.
The aforementioned behavior can be observed in ALZ (with – OH substituents group acting as electron donor by mesomeric effect) and MDA (with – CH3 group acting as electron donor by inductive effect), and this can be attributed to the dislocation of the reduction potential to more negative values (potential/mV), leading to an increase in the amount of H2O2 generated in situ. Based on the chemical structure shown in Fig. 2, the presence of − OH and − CH3 groups (electron donor) in ALZ and MDA modifiers is responsible for the increase observed in the reduction ability of the compounds in comparison to AQ (without substituents) [23].
With regard to ALZ, the proximity of the − OH group (H donor center) with the CO portion (H acceptor center) facilitates the formation of intramolecular bonds (hydrogen bonding) in such a way as to stabilize the radical anion intermediate formed during the process (see the extended mechanism). As ALZ possesses 2 groups or − OH centers, it, thus, exhibits a higher reduction ability and capability of generating more H2O2 in situ compared to MDA (only 1 electron-donating inductive center or − CH3 group).
Finally, it is worth noting that the structure of APQ differs from that of ALZ and MDA. APQ has no electron-donating substituents, and its reduction capacity with potential for H2O2 generation is less pronounced than the others, as can be observed in Eqs. 11 to 14.
Figure 4 shows the results obtained in terms of H2O2 production for carbon modified with 1% ALZ, carbon modified with other compounds, and for unmodified carbon. The modification of carbon with 1% ALZ led to the highest production of H2O2. This behavior may be associated with the mechanism of oxygen reduction, directing the reaction toward 2 electrons, leading to the formation of H2O2. In this sense, the constant current experiments provided quantified energy values. However, based on the modifier employed, different amounts of H2O2 are produced. This difference is associated with the amount of energy directly applied to the H2O2 generation reaction (Eqs. 3 and 4) and the amount of energy used in the reactions that occur parallel to the oxygen reduction reaction (Eqs. 5 and 6).
The electrical efficiency (EE%) shows the relation between the electric charge for quantified H2O2 and the total electric charge in each experiment.
To determine the electrical efficiency (Eq. 15), the number of moles of quantified H2O2 (H2O2R: mg L−1 converted to moles) obtained in Fig. 4 was calculated taking into account the electrolyte volume in each experiment (400 mL). Equation 14 was used to calculate the theoretical number of moles of H2O2 (H2O2T); this value was obtained from the total electrical charge applied (10–100 mA cm−2 for 90 min) considering that all the electrical charge was used for H2O2 generation (based on Eq. 15. The use of H2O2T is linked to the fact that the electrochemical cell has a single compartment and one is unable to determine the partial currents of the electrochemical reactions that occur simultaneously. In this sense, EE% shows how the total electrical charge (Eq. 14) was effectively applied to obtain the quantified H2O2 presented in Fig. 4.
Figure 5 shows the electrical efficiency (EE%) values related to H2O2 generation for the materials modified with 1% of each of the organic compounds investigated. As can be observed, lower values of applied current density presented higher values of electrical efficiency, while an increase in applied current value resulted in a decrease in the electrical efficiency of H2O2 generation. This behavior is attributed to the amount of energy supplied to the reduction reactions. When the energy is small, most of it is used for the reduction of oxygen via the 2-electron mechanism; however, when there is an increase in the energy supplied (increase in the applied current density), part of the energy is used in the reduction reactions which occur simultaneously (Eqs. 5 and 6), decreasing the electrical efficiency of H2O2 generation.
Carbon modification with ALZ promoted a higher electrical efficiency, reaching 25.5% of the current at 10 mA cm−2 applied directly to the reaction involving H2O2 generation, while the experiment with 150 mA cm−2 reached only 5.1%. The decrease observed in electrical efficiency may be associated with the excess energy generated, which contributed to an increase in the reactions parallel to the H2O2 generation reaction.
Conclusions
The present study has shown that the use of quinones as carbon black modifier is efficient for H2O2 generation in acid medium. With regard to O2 reduction current and H2O2 detection current, a comparison of the carbon electrode modifiers showed that 1,2-dihydroxyanthraquinone presented the best current values, reaching 95% efficiency for H2O2 detection current with an exchange of 2.1 electrons. Analyses of H2O2 generation on GDE showed that the use of 1,2-dihydroxyanthraquinone contributed to the highest H2O2 generation, where the amount of 289 mg L−1 of H2O2 was obtained at the end of 90 min of treatment for the electrodes modified with 1% of 1,2-dihydroxyanthraquinone.
The results show that the compounds related to the quinone group, 1% of 1,2-dihydroxyanthraquinone, are efficient for H2O2 generation on gas diffusion electrodes based on the application of the electrochemical/chemical mechanism, where the chemical structure of the modifier directly influences the amount of H2O2 generated.
References
S. Ranganathan, V. Sieber, Catalysts 8, 379 (2018)
R.B. Valim, R.M. Reis, P.S. Castro, A.S. Lima, R.S. Rocha, M. Bertotti, M.R.V. Lanza, Carbon 61, 236 (2013)
J.M. Campos-Martin, G. Blanco-Brieva, J.L.G. Fierro, Angew. Chem. Int. 45, 6962 (2006)
E.L. Gyenge, C.W. Oloman, J. Appl. Electrochem. 33, 655 (2003)
D. Bao, S. Ramu, A. Contreras, S. Upadhyayula, J.M. Vasquez, G. Beran, V.I. Vullev, J. Phys. Chem. B 114, 14467 (2010)
J.-S. Do, C.-P. Chen, J. Appl. Electrochem. 24, 936 (1994)
D. Alvarez-Gallegos, Pletcher. Electrochim. Acta 44, 853 (1998)
C. Badellino, C.A. Rodrigues, R. Bertazzoli, J. Appl. Electrochem. 37, 451 (2007)
S.C. Perry, D. Pangotra, L. Vieira, L.-I. Csepei, V. Sieber, L. Wang, C.P. de Leon, F.C. Walsh, Nat. Rev. Chem 3, 442 (2019)
E. Brillas, M.A. Banos, S. Camps, C. Arias, P.L. Cabot, P.L. Garrido, J.A.R.M. Rodríguez, New J. Chem. 28, 314 (2004)
H.W. Kim, M.B. Ross, N. Kornienko, L. Zhang, J. Guo, P. Yang, B.D. McCloskey, Nat. Catalysis. 1, 282 (2018)
C.A.R. Ragnini, R.A. Di Iglia, R. Bertazzoli, Quim Nova 24, 252 (2001)
M. Giomo, A. Buso, P. Fier, B. Boye, G.A. Farnia, Electrochim Acta 54, 808 (2008)
M.H.M.T. Assumpção, A. Moraes, R.F.B. De Souza, I. Gaubeur, R.T.S. Oliveira, V.S. Antonin, G.R.P. Malpass, R.S. Rocha, M.L. Calegaro, M.R.V. Lanza, M.C. Santos, Appl. Catal. A Gen. 411-412, 1 (2012)
R. Abdel-Hamid, M.K. Rabia, H.M. El-Sagher, Bull. Chem. Soc. Jpn. 70, 2389 (1997)
S. Komorsky-Lovric, J. Anal. Chem. 356, 306 (1996)
X.-S. Chai, Q.X. Hou, Q. Luo, J.Y. Zhu, Anal. Chim. Acta 507, 281 (2004)
P.S. Guin, S. Das, P.C. Mandal, Int. J. Electrochem. 189, 206 (2011)
J.F. Carneiro, R.S. Rocha, P. Hamer, R. Bertazzoli, M.R.V. Lanza, Appl. Catal. A: General. 517, 161 (2016)
L.R. Radovic, A.J.A. Salgado-Casanova, Carbon. 126, 443 (2018)
A.M.O. Brett, C.M.A. Brett, Electrochemistry: Principles, Methods and Applications, 5nd edn. (Oxford University Press 1996), p. 273
M.H.M.T. Assumpção, R.F.B. De Souza, D.C. Rascio, J.C.M. Silva, M.L. Calegaro, I. Gaubeur, T.R.L.C. Paixão, P. Hammer, M.R.V. Lanza, M.C. Santos, Carbon. 49, 2842 (2011)
K. Tammeveski, K. Kontturi, R.J. Nichols, R.J. Potter, D.J. Schiffrin, J. Electroanal. Chem. 515, 101 (2001)
P. Huissoud, J. Tissot, Appl. Electrochem. 28, 653 (1998)
F. Wang, S. Hu, Electrochim. Acta 51, 4228 (2006)
Y. Feng, T. He, N.A. Vante, Electrochim. Acta 54, 5252 (2009)
E. Roche, M. Chainet, J. Chatenet, J. Vondrak, Phys. Chem. C 111, 1434 (2007)
R.M. Reis, A.A.G.F. Beati, R.S. Rocha, M.H.M.T. Assumpção, M.C. Santos, R. Bertazzoli, M.R.V. Lanza, Ind. Eng. Chem. Res. 51, 649 (2012)
Funding
The authors are sincerely grateful to the Brazilian research funding agencies, including the Brazilian National Council for Scientific and Technological Development – CNPq (grants no. 465571/2014-0, 302874/2017-8 and 427452/2018-0), São Paulo Research Foundation (FAPESP – grants #2011/14314-1, #2016/22115-2, #2014/50945-4, #2013/02762-5, #2016/12597-0, #2019/00239-0 and #2017/10118-0), and the Coordenação de Aperfeiçoamento de Pessoal de Nível Superior (CAPES – Finance Code 001) for the financial support granted in the course of this research.
Author information
Authors and Affiliations
Corresponding authors
Additional information
Publisher’s Note
Springer Nature remains neutral with regard to jurisdictional claims in published maps and institutional affiliations.
Rights and permissions
About this article
Cite this article
Rocha, R.S., Valim, R.B., Trevelin, L.C. et al. Electrocatalysis of Hydrogen Peroxide Generation Using Oxygen-Fed Gas Diffusion Electrodes Made of Carbon Black Modified with Quinone Compounds. Electrocatalysis 11, 338–346 (2020). https://doi.org/10.1007/s12678-020-00591-1
Published:
Issue Date:
DOI: https://doi.org/10.1007/s12678-020-00591-1