Abstract
To evaluate the important odor components in raw yellowtail, volatiles from each part of stored yellowtail flesh were analyzed using gas chromatography-mass spectrometry (GC–MS) and olfactometry (GC–O). The principal component analysis (PCA) results from GC–MS of 88 volatiles showed that PC1 separated the data into two groups [the dark muscle (DM) long storage] and [the ordinary muscle (OM) + DM no storage + DM short storage]. Eleven compounds, including 2,3-butanedione, in 24 kinds of odors were identified or tentatively estimated using GC–O analysis. The numbers of odors perceived at the maximum split ratio (243) in GC–O analysis were 8 and 12 from OM and DM, respectively, irrespective of storage time. Kovats retention index 1387 (unknown compound, fishy and plastic odor) had the strongest odor in both muscles before and after storage. GC–O analysis showed that DM odors were more intense than those from dorsal OM. Moreover, their intensities increased during storage. Sensory evaluation revealed that DM had a stronger odor than OM throughout the storage period. These findings suggested that odor components, which showed small thresholds, as well as other identified components from DM, such as 2,3-butanedione, contributed to the yellowtail meat odor before and after storage.
Similar content being viewed by others
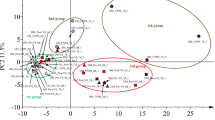
Explore related subjects
Discover the latest articles, news and stories from top researchers in related subjects.Avoid common mistakes on your manuscript.
Introduction
Fish flesh is rich in high-quality protein and in n-3 polyunsaturated fatty acids (PUFAs), such as eicosapentaenoic acid, and docosahexaenoic acid. Dark muscle (DM) in fish flesh, in particular, is abundant in various bioactive and nutritious compounds such as glutathione, heme iron, and taurine, in addition to the abovementioned compounds (Song et al. 2000; Sohn et al. 2005; Sohn et al. 2007; Tanimoto et al. 2011). Hence, fish meat is recommended for maintaining a healthy lifestyle. However, fish meat deteriorates easily during storage and processing. Odor change is one of the most influential factors in degrading the quality of fish. Some individuals tend to not eat fish-based cuisine due to its pungent odor.
The yellowtail fish, Seriola quinqueradiata, is important for aquaculture in Japan. The production of this fish, which is farmed in southern parts of Japan, was 140,292 tonnes in 2015 (Production, Marketing and Consumption Statistics Division, Statistics Department, Minister’s Secretariat, Ministry of Agriculture, Forestry and Fisheries, Kaimen Gyogyou Toukeichousa, sea fishery statistics: http://www.e-stat.go.jp/SG1/estat/List.do?lid=000001167223/ "accessed 4 August 2017"). The fish is produced for domestic consumption, as well as for export. The Japanese eat yellowtail meat in its raw and cooked state. Ordinary muscle (OM) from yellowtail, individually or in combination with DM, is used in Japanese cuisine. The quality of fish meat, especially that of raw meat, is important for consumer acceptability.
Many studies have examined changes in the volatile compounds during storage in various fish types (Kawai 1996; Ganeko et al. 2008; Miyasaki et al. 2011; François et al. 2012; Moreira et al. 2013; Foteini et al. 2014). Several carbonyl compounds are secondary oxidation products from action of lipoxygenase or auto-oxidation of PUFAs. These volatiles might be involved in imparting the characteristic fishy odor to fish flesh (Josephson et al. 1984). Trimethylamine is another contributor to the odor of seawater fish (Koizumi et al. 1979). However, Ganeko et al. (2008) and Miyasaki et al. (2011) reported that carbonyl compounds, rather than trimethylamine, contribute to the fishy odor of fish flesh. However, most of abovementioned studies were carried out on OM or fish flesh from which DM was not removed. Although we have previously studied changes in the volatile compounds in OM as well as DM of yellowtail during short-term cold storage (Tanimoto and Shimoda 2016), there has been limited information regarding volatile compounds in DM (Tokunaga et al. 1981; Hiratsuka et al. 2011). In particular, volatile compounds that contribute to odors in DM and OM of yellowtail before and after cold storage remain unclear.
The objective of this study was to evaluate the important odor components in both OM and DM of yellowtail fish flesh before and after cold storage, using solid phase microextraction (SPME), followed by GC–MS and olfactometry (GC–O). We also measured visible cell counts, color, two indices of oxidation [thiobarbituric acid-reactive substances (TBARS) and peroxide value (PV)], and fatty acid composition, and evaluated the sensory characteristics of yellowtail fish meat to investigate the relationship between flavor components (volatile compounds) and these parameters during cold storage.
Materials and methods
Experimental animals
Six cultured yellowtail fish, Seriola quinqueradiata were purchased from a local market in Hiroshima, Japan. The average weight of these fish was 5.4 ± 1.2 kg. The fish were transported to the laboratory on ice within 8 h of being killed at the market and then filleted. Left and right fillets of each fish were used in the study. The fillets from two fish were rinsed with chilled 1% saline, and were separated according to each part of the flesh (OM: dorsal flesh, ventral flesh, caudal flesh, and DM). Each part was minced using a food processor (MK-K60P, Panasonic, Kadoma, Osaka, Japan). The minced flesh was wrapped with a polyethylene film to be shaped into thin plates (9.5 × 7.0 × 1.0 cm) without headspace to prevent the surface from drying. The plates were stored in ice for 14 days or at 5 °C for 7 days. At 0, 3, 7, and 14 days of storage in ice, and at 1, 3, and 7 days of storage at 5 °C, each sample was frozen in a blast chiller/freezer (iRiNOX AL-5 M, FMI, Osaka, Japan) and stored at − 80 °C until analyses, with the exception of psychrophilic viable cell count and color measurement. This process was repeated 3 times. Three samples from each part of the flesh were prepared in this study (n = 3).
Viable cell counts
Each part of the flesh (5 g) from three replicates was homogenized with 45 ml of 0.85% physiological saline on the day of sampling. The homogenates were serially diluted using the same solution. Viable cell counts were determined by plating 1-ml aliquots of diluted or undiluted samples on plates of standard agar medium (0.25% yeast extract, 0.5% polypeptone, 0.1% glucose, 1.5% agar, and final pH 7.1). The plates were aerobically incubated at 35 °C for 48 ± 3 h. Colonies were counted after the incubation. Viable cell counts were expressed as colony forming units per sample weight (cfu/g).
Color measurements
The browning of DM was evaluated by measuring the b/a ratio on sampling day (Hiraoka et al. 2004). The a (redness/greenness) and b (yellowness/blueness) values were measured using a colorimeter (CR-400; Konica Minolta Optics, Inc., Tokyo, Japan). An average of six measurements on the surface of a minced DM was used for calculating the average from three replicate samples.
Determination of TBARS values
A frozen sample (0.50–2.50 g) from three replicates was homogenized in an appropriate volume (2- to 64-fold volume) of 1.15% KCl solution (w/v) on ice using a glass/Teflon Potter–Elvehjem tissue homogenizer. The homogenate (200 μl) was mixed with 650 μl TBA-EDTA solution (0.20 ml of 5.2% SDS solution (w/v), 50 μl of 0.8% dibutylhydroxytoluene in acetate (w/v), 1.50 ml of 0.80% thiobarbituric acid solution (w/v), 1.70 ml of 3.0 mM EDTA), and 150 μl of 3.40 M sodium acetate buffer (pH 3.5) in a screw-capped test tube. This mixture was kept on ice for 1 h. The tube was heated in boiling water for 60 min and immediately cooled to room temperature. One milliliter of n-butanol-pyridine mixture (30:2) was vortexed with the reaction mixture. After centrifugation at 700×g for 10 min, absorbance of the butanol layer was measured at 532 nm. The concentrations of TBARS in the flesh samples were expressed as nmol malondialdehyde/g flesh using 1,1,3,3-tetramethoxypropane as the standard.
Total lipid content
Lipids were extracted using chloroform/methanol (2:1), according to the method given by Bligh and Dyer (1959). A frozen sample (2.5 g of DM and 5.0 g of OM) from three replicates was used for analysis. The total lipid content was determined gravimetrically.
Determination of PV
PV of the total lipid fraction from fish flesh was analyzed using a diphenyl-1-pyrenylphosphine-based fluorometric method with a slight modification (Akasaka et al. 1992). PV of corn oil, as determined by iodometry, was used as a standard for calculation of PV (AOCS 1960). This value was expressed as mmol/kg total lipid.
Fatty acid composition
The total lipid fraction was converted to fatty acid methyl ester by direct trans-esterification using methanol containing 5–10% hydrochloric acid (Tokyo Kasei Kogyo Co. Ltd., Tokyo, Japan). The composition of the fatty acid esters was determined from gas chromatography using a Shimadzu GC 14A (Shimadzu, Kyoto, Japan), equipped with a flame ionization detector (GC–FID). Separation was achieved on an InertCap WAX capillary column (30 m × 0.25 mm, 0.25-μm film thickness, Tokyo, GL sciences, Inc.) under conditions described below. The temperature was held at 50 °C for 5 min, then raised at a rate of 10 °C/min to 250 °C, and held for 15 min. The temperature was further increased at a rate of 10 °C/min to 260 °C with a final hold time of 10 min. The injection port and detector were maintained at 250 and 260 °C, respectively. Helium was used as the carrier gas with a constant inlet head pressure of 100 kPa. The injection was conducted in split mode (split ratio, 10).
SPME of volatile compounds
A frozen sample (1.5 g) from three replicates was homogenized in 7.5 ml saturated NaCl solution on ice, using a glass/Teflon Potter–Elvehjem tissue homogenizer. This saturated solution was used to accelerate transfer of volatile compounds to the vial headspace by salting-out effect. The homogenate was centrifuged at 10,000×g for 15 min at 0 °C. The supernatant (5.0 ml) was mixed with 5 μl of 0.010% cyclohexanol solution (internal standard) in a 20-ml vial. The vial was tightly capped with a polypropylene holed-cap with a polytetrafluoroethylene/silicone septum and incubated in a water bath for 60 min at 40 °C. After incubation, a SPME fiber (Supelco Co., Bellefonte, PA, USA) coated with 75 μm of carboxen-polydimethylsiloxane was exposed in the vial headspace for 30 min at 40 °C. After extraction, the fiber was retrieved and placed in the injection port of a GC–MS or a GC–FID for sampling.
Gas chromatography–mass spectrometry
Volatile compounds were analyzed using a Shimadzu GCMS-QP2010 mass spectrometer (Shimadzu, Kyoto, Japan). Separation was carried out on a DB-WAX capillary column (30 m × 0.25 mm, 0.5-μm film thickness, Agilent Technologies, Inc., Santa Clara, CA, USA). Separation was achieved as follows. The temperature was held at 35 °C for 10 min, then raised at a rate of 4 °C/min to 230 °C. The mass spectrometer was operated in the electron-impact ionization (EI) mode. The temperature of the GC–MS transfer line was 200 °C. The ionization voltage was set at 70 eV. The mass spectrometer scanned masses from 40 to 300 m/z at a rate of 2.0 scan/s. The Kovats retention index (KI) was determined using a homologous series of n-alkanes (C9–19) (Kovats 1965):
where n is the number of carbon atoms in reference n-alkane; t x , the retention time of a volatile compound, and t n and t n+1, are the retention times of n-alkanes which elute immediately before and after the volatile compound (x). The compounds were identified by comparison with mass spectra from NIST/EPA/NIH Mass Spectral Library (NIST 11) (National Institute of Standards and Technology, Gaithersburg, MD, USA) and the Kovats retention index. The injection port was maintained at 230 °C. Helium was used as the carrier gas with a constant inlet head pressure of 50 kPa. Injection was conducted in splitless mode for 1.0 min with simultaneous cryofocusing with liquid nitrogen and in a split mode for 5.0 min (split ratio, 10). The semi-quantitative value of each volatile in each fish sample (ng/g) was estimated by using the ratio of total ion peak area of the volatile to that of the internal standard (0.010% cyclohexanol solution).
Gas chromatography–flame ionization detector/olfactometry (GC–FID/O)
Odor compounds were analyzed using a Shimadzu GC 2014 (Shimadzu), equipped with FID (Shimadzu) and sniffing port (OP275, GL sciences, Inc.). A DB-WAX capillary column (30 m × 0.25 mm, 0.5-μm film thickness, Agilent Technologies) was used for separation. The inlet head pressure of the carrier gas was constant at 100 kPa. The detector was maintained at 240 °C. The split ratio was set at 1, 3, 9, 27, 81 or 243 to evaluate intensity of the odor compound. Other chromatographic conditions for the GC–FID/O analysis were same as those for the GC–MS analysis. The effluent from the capillary column was split 1:1 (v/v) between the FID and the sniffing port using a “Y” splitter. A panel of two trained assessors (female, undergraduate and graduate students of the Prefectural University of Hiroshima, aged 23 and 24) performed GC–O sniffing analysis. The assessors decided the retention time and odor characteristics when an odor was perceived.
Sensory evaluation
Sensory evaluation was conducted by 33 trained panelists (2 males and 31 females aged from 21 to 23 years; all were undergraduate and graduate students of the Prefectural University of Hiroshima). The scaling method using a 5-point scale (1, very weak; 2, weak; 3, normal; 4, strong; 5, very strong) was used for the evaluation. The panel judged the intensities of overall, fishy, and oily odor of each part of the raw fish flesh before storage and after 7 days of storage at 0 °C. A frozen sample (5 g) was put into a vial (20 ml) and thawed on ice. After 1 h, the sample was evaluated.
Statistical analyses
Student’s t-test and Tukey’s multiple-range test were applied to determine differences between the mean values of samples. The analyses were performed using SPSS Statistics 17.0 (IBM Japan, Ltd., Tokyo, Japan). The significance level was set at 5%. Principal component analysis (PCA) was performed with Pirouette 4.5 (Infometrix, Inc., Bothell, WA, USA) to analyze the data of volatile compounds from GC–MS.
Results
Viable cell counts and color
Figure 1 indicates the changes in viable cell counts of yellowtail fish flesh during storage. After 7 days of storage at 5 °C, viable cells in OM of the ventral flesh increased significantly (P < 0.05), but the increase in magnitude was only one order, as compared to its initial values. The other yellowtail flesh at 5 °C and all flesh at 0 °C showed no significant increment during storage. There were no significant differences among viable cell counts in the flesh of yellowtail fish after the same storage times. Figure 2 indicates the changes in b/a values of DM during storage at 0 and 5 °C. The b/a values of DM after 7 days of storage at 0 °C and 5 °C were significantly higher than those before storage (P < 0.05). Therefore, although browning (metmyoglobin formation) of yellowtail DM occurred during storage, no microbial action was observed in yellowtail fish flesh under the storage conditions in the present study.
Microbiological changes in yellowtail flesh at 0 °C (left), and 5 °C (right) during storage. OD ordinary muscle in dorsal part, OV ordinary muscle in ventral part, OC ordinary muscle in caudal part, DM dark muscle. Error bars represent standard deviation for the sample triplicates (n = 3). Different letters for OV stored at 5 °C indicate significant differences (P < 0.05). There is no significant difference among different types of flesh at the same storage time
Lipid oxidation and fatty acid composition
Table 1 indicates the changes in TBARS, PV, and total lipid content of yellowtail flesh during storage at 0 and 5 °C. Levels of TBARS in yellowtail DM at the same storage time, except on day 3, were significantly higher (P < 0.05) at both storage temperatures, as compared with those in the other flesh. TBARS values of DM were significantly higher than those before storage (P < 0.05) at 14 and 7 days of storage at 0 °C and 5 °C, respectively. TBARS levels of the OM samples were not significantly different after storage, as compared to the fresh samples, except for ventral flesh after day 7 of storage at 0 °C and dorsal flesh at day 7 of storage at 5 °C. In contrast, there were no significant differences among the PVs in each type of yellowtail flesh before storage and after the same storage time. PV in ventral flesh at day 14 of storage at 0 °C and at day 7 of storage at 5 °C and in dorsal flesh at day 7 of storage at 5 °C were significantly higher than those before storage (P < 0.05). The total lipid contents of caudal flesh of OM were significantly lower than those of the ventral flesh of OM and of the DM at the same storage time and temperature. The total lipid content in each type of yellowtail flesh did not change significantly during storage at either temperature.
Table 2 shows the fatty acid composition of yellowtail fish flesh at day 0 and day 14 of storage at 0 °C. The fatty acid composition of each type of yellowtail flesh at the same storage time and temperature was not significantly different from each other, except for a part of the fatty acid species (Supplementary data Tables S1 and S2). In addition, percentages of all fatty acid species of each type of yellowtail flesh did not change significantly during storage at both temperatures.
Volatile compounds
Table 3 shows the volatile compounds in yellowtail fish flesh after storage at 0 and 5 °C. During storage, 88 compounds (26 aldehydes, 22 alcohols, 12 ketones, 9 alkanes, 5 carboxylic acids, 5 furans, 2 esters, 2 nitrogen-containing compounds, 2 sulfur-containing compounds, 1 lactone, and 2 other compounds) were identified in the flesh (Supplementary data Tables S4–S7). The levels of many volatile compounds were significantly higher (P < 0.05) in DM than in other types of flesh at the same storage time under both storage temperatures. Although the number of volatile compounds, which had significantly higher content in DM, as compared to other types of flesh (P < 0.05), was 5 before storage, it changed to 45 after 14 days of storage at 0 °C and to 27 after 7 days of storage at 5 °C. The number of volatile compounds, which increased significantly during storage (P < 0.05), was 10–15 in OM and 40 in DM at 0 °C and 2–7 in OM and 31 in DM at 5 °C. These results indicated that changes in volatile compounds in yellowtail fish meat mainly developed in DM.
The results of PCA of volatile compounds from GC–MS in yellowtail fish meat during storage at 0 and 5 °C are shown in Figs. 3 and 4. The scores for the first 2 principal components (PC1 and PC2) accounted for 41.9% and 11.7% of the variation, respectively. PC1 separated the data into two groups: (DM long storage) and (OM + DM no storage + DM short storage). In contrast, each part of OMs with longer storage time showed higher PC2 scores. Many volatile compounds such as 2-methyl furan had high-factor loadings for PC1 (Fig. 4 and Supplementary data Table S7). These volatiles contributed to the change in DM during storage and to the difference between OM and DM with longer storage time for PC1. For PC2, alcohols, such as hexanol and 1-octanol, had high-factor loadings, but aldehydes, such as hexanal and pentanal, had low-factor loadings. Therefore, changes in these compounds were involved in the changes in PC2 scores of OM during storage.
Scatter plot of principal components (PC1 and PC2) for yellowtail fish flesh during storage at 0 and 5 °C by using the peak areas of 88 volatile compounds. Symbols are the same as in Fig. 1. Storage temperature and time of each flesh are indicated as follows: example OD_3_0, storage for 3 days at 0 °C
Loading plot of principal components (PC1 and PC2) for yellowtail fish flesh during storage at 0 and 5 °C using peak areas of 88 volatile compounds. The numbers indicate the Kovats index of volatile compounds in Table 3
GC–O analysis
Table 4 presents volatile components obtained via GC–O analysis of yellowtail fish flesh at day 0 and day 7 of storage at 0 °C. Twenty-four kinds of odors were sensed using GC–O analysis. In these odors, eight compounds were identified by GC/MS and Kovats index, and three compounds were tentatively estimated by odor characteristic and Kovats index of authentic samples. An unknown compound (KI 1387) showed the strongest odor in both muscles before and after storage. The numbers of odors perceived at the maximum split ratio (243) were 8 and 12 from OM in dorsal part and DM, under both storage conditions, respectively, indicating that the odor of yellowtail DM was stronger than that of OM from GC–O. Low boiling point odors, which are low Kovats index, from DM had a larger split ratio before storage, as compared to those on day 7 of storage. However, high boiling point odors showed contrasting results. The high boiling point odors from DM after 7 days of storage were sensed more strongly, as compared to the low boiling point odors before storage. Therefore, the odors sensed in yellowtail DM using GC–O have stronger intensity, compared with those in OM in the dorsal part, and their intensities were enhanced during storage.
Sensory evaluation
Figure 5 shows the results of the sensory evaluation of the odor of yellowtail fish flesh at day 0 and day 7 of storage at 0 °C. The sensory scores of DM from all evaluated items were significantly higher than those from other parts of the flesh under the same storage conditions (P < 0.05). In addition, odor intensity and fishy odor of DM were enhanced significantly after 7 days of storage (P < 0.05). However, the odor intensities of OM in dorsal and ventral parts decreased significantly after 7 days of storage (P < 0.05). Therefore, the odor of DM was stronger than that of OM throughout the storage period. Although the odor level of DM increased during storage, that of OM decreased under the same storage conditions.
Sensory test of yellowtail fish flesh stored for 0 and 7 days at 0 °C. OD ordinary muscle in dorsal part, OV ordinary muscle in ventral part, OC ordinary muscle in caudal part, DM dark muscle. Capital letters at the same storage time with a different letter indicate significantly different values (P < 0.05). Small letters for the same flesh with a different letter indicate significantly different values (P < 0.05)
Discussion
Visible cell counts in most parts of yellowtail flesh showed no significant increase during storage (Fig. 1). Tanimoto and Shimoda (2016) also reported that viable cells in OM remained at the same order of magnitude as their initial values for 2 days at 5 °C. These results indicate that qualitative changes, such as content of volatiles, in yellowtail meat proceeded without any microbial action.
The TBARS values of DM were significantly higher than those of other parts of yellowtail flesh, and increased significantly during storage (P < 0.05, Table 1). Though PVs of some parts of yellowtail flesh increased during storage (P < 0.05), PVs from each part of yellowtail flesh did not differ significantly from each other on the same day of storage. These results suggested that lipid hydroperoxide in DM degraded rapidly to secondary oxidation products during storage. With certain exceptions, OM in the ventral flesh showed no significant difference in the total lipid contents, and showed significantly lower TBARS (P < 0.05) as compared to those of the DM at the same storage time and temperature (Table 1). A reason for this phenomenon could be that myoglobin (heme iron), which was abundant in DM, accelerated the lipid oxidation.
Many researchers have reported that various volatiles in fish meat increased during storage (Kawai 1996; Ganeko et al. 2008; Miyasaki et al. 2011; François et al. 2012; Moreira et al. 2013; Foteini et al. 2014). In this study, several volatile compounds in yellowtail flesh also increased significantly during storage (P < 0.05, Table 3, Supplementary data Tables S3–S6). In addition, the number of volatile compounds, which increased significantly during storage, was larger in DM than in other parts of flesh (P < 0.05). Alcohols, such as 1-penten-3-ol and 1-pentanol, aldehydes, such as (Z)-4-heptenal and (E,Z)-2,4-heptadienal, and ketones, such as 1-penten-3-one, increased significantly in DM during storage. François et al. (2012) reported that hexanal level in European seabass decreased after 1, 4, and 15 days of storage on ice. The hexanal level in OM of each flesh part decreased significantly during storage in this study (P < 0.05, Table 3, Supplementary data Tables S3–S6). However, hexanal, which was generated by oxidative decomposition of unsaturated fatty acids (Moreira et al. 2013), in DM increased significantly during storage. In our previous paper, many volatile compounds detected in DM of yellowtail increased significantly during 2 days of storage, and increased significantly in OM after 1 day of storage, but decreased or remained at the same level during the subsequent storage period (Tanimoto and Shimoda 2016), supporting results from the present study. The number of volatile compounds, [which had significantly higher contents in DM, as compared to those in the other parts of flesh at the same storage time under both storage temperatures (P < 0.05, Table 3, Supplementary data Tables S3–S6)] was large both before and after storage. These numbers also increased during storage. These results indicated that the changing patterns of volatile compounds in yellowtail flesh were different between DM and OM. However, 2-methyl-pentanol and 1-hexanol increased significantly in both muscles. Therefore, the content of these volatiles might be proposed as a quality index for yellowtail during cold storage.
The results of GC–O showed that the number of odors, which were sensed at the maximum split ratio (243), was larger in DM than in OM in the dorsal flesh at both storage times (Table 4). These odors in DM were stronger than those in OM. In addition, the intensities of many odors sensed in DM after 7 days of storage were stronger than each intensity level before storage. In contrast, many odors sensed in the OM of the dorsal flesh after 7 days of storage decreased in their intensities, as compared to those before storage. These results were supported by the changing profile of the volatile compounds in DM and OM in the dorsal flesh during storage (Table 3, Supplementary data Tables S3–S6) and by the results of sensory evaluation (Fig. 5) in this paper. Increase in the odor intensity of 1-octen-3-one (mushroom-like, fungus-like, and fishy) in DM, and the decrease of the same in the OM in the dorsal flesh after storage (Table 4) were coincident with fluctuation of this volatile during storage (Table 3, Supplementary data Tables S3–S6) and results of sensory evaluation (Fig. 5), suggesting that this compound contributed to the odor of yellowtail meat. Though the hexanal level of OM in the dorsal flesh significantly decreased during storage, the intensity of odor sensed at the same KI value in this part increased after storage (Table 4). This discrepancy might mean that other compounds, which have a very small threshold, were co-eluted with hexanal at the same retention time. François et al. (2012) also reported a decrease in the hexanal level and an increase in the odor intensity at the same linear retention indices as this compound in European seabass during storage. This result was consistent with our present study. The compounds 2,3-butanedione, 2,3-pentadione, hexanal, 1-octen-3-one, and (E,E)-2,4-heptadienal were sensed at the maximum split ratio (243). However, several odors, which were sensed at this split ratio, were not detected by GC/MS (Table 4). These compounds were considered to have very small thresholds. The number of odors with higher KI value and the maximum split ratio was specifically higher in DM, as compared to the OM in the dorsal flesh before and after storage (Table 4). This suggests that these odors were highly involved in the DM odor of yellowtail. In addition, these odors from DM after 7 days of storage had higher intensities than those before storage.
In conclusion, these findings suggested that odor components, which had very small thresholds, from DM contributed to the change in odor of yellowtail meat during storage, as compared to the change in odor of OM.
References
Akasaka K, Sasaki I, Ohrui H, Meguro H (1992) A simple fluorometry of hydroperoxides in oils and foods. Biosci Biotechnol Biochem 56:605–607
AOCS (1960) Method Cd 8-53. In: Official and tentative method of the American Oil Chemists’ Society, Vol. I & II, 3rd edn. American Oil Chemists’ Society, Champaign, IL, USA
Bligh EG, Dyer WJ (1959) A rapid method for total lipid extraction and purification. Can J Biochem Physiol 37:911–917
Foteini FP, Athanasios M, Serkos AH, Ioannis SB (2014) Microbiological spoilage and investigation of volatile profile during storage of sea bream fillets under various conditions. Inter J Food Microbiol 189:153–163
François L, Pascal T, Ko Nathalie K, Frédéric M, Pierre M, Ossarath K, Jean LB, Guillaume D (2012) Evolution of volatile odorous compounds during the storage of European seabass (Dicentrarchus labrax). Food Chem 131:1304–1311
Ganeko N, Shoda M, Hirohara I, Bhadra A, Ishida T, Matsuda H, Takamura H, Matoba T (2008) Analysis of volatile flavor compounds of sardine (Sardinops melanostica) by solid phase microextraction. J Food Sci 73:S83–S88
Hiraoka Y, Ohsaka E, Narita K, Yamabe K, Seki N (2004) Preventive method of color deterioration of yellowtail dark muscle during frozen storage and post thawing. Fish Sci 70:1130–1136
Hiratsuka S, Aoshima S, Koizumi K, Kato N (2011) Changes of the volatile flavor compounds in dark muscle of skipjack tuna during storage. Nippon Suisan Gakkaishi 77:1089–1094 (in Japanese with English abstract)
Josephson DB, Lindsay RC, Stuiber DA (1984) Biogenesis of lipid-derived volatile aroma compounds in the emerald shiner (Notropis atherinoides). J Agric Food Chem 32:1347–1352
Kawai T (1996) Fish flavor. Crit Rev in Food Sci Nutr 36:257–298
Koizumi C, Kieu-Thu thi C, Nonaka J (1979) Undesirable odor of cooked sardine meat. Nippon Suisan Gakkaishi 45:1307–1312 (in Japanese with English abstract)
Kovats ES (1965) Gas chromatographic characterization of organic substances in the retention index system. Adv Chromatogr 1:229–247
Miyasaki T, Hamaguchi M, Yokoyama S (2011) Change of volatile compounds in fresh fish meat during ice storage. J Food Sci 76:C1319–C1325
Moreira N, Valente LMP, Castro-Cunha M, Cunha LM, Pinho PG (2013) Effect of storage time and heat processing on the volatile profile of Senegalese sole (Solea senegalensis Kaup, 1858) muscle. Food Chem 138:2365–2373
Sohn JH, Taki Y, Ushio H, Kohata T, Shioya I, Ohshima T (2005) Lipid oxidations in ordinary and dark muscles of fish: influences on rancid off-odor development and color darkening of yellowtail flesh during ice storage. J Food Sci 70:S490–S496
Sohn JH, Ushio H, Ishida N, Yamashita M, Terayama M, Ohshima T (2007) Effect of bleeding treatment and perfusion of yellowtail on lipid oxidation in post-mortem muscle. Food Chem 104:962–970
Song XA, Hirata T, Sakaguchi M (2000) Proximate composition and nitrogenous extractive components in fish muscle and internal organ. Nippon Suisan Gakkaishi 66:282–290 (in Japanese with English abstract)
Tanimoto S, Shimoda M (2016) Changes in volatile compounds of dark and ordinary muscles of yellowtail (Seriola quinqueradiata) during short-term cold storage. J Aquat Food Prod Technol 25:185–196
Tanimoto S, Song XA, Sakaguchi M, Sugawara T, Hirata T (2011) Levels of glutathione and related enzymes in yellowtail fish muscle subjected to ice storage in a modified atmosphere. J Food Sci 76:C974–C979
Tokunaga T, Iida H, Nakamura K, Ota Y (1981) Changes of the volatile components in round mackerel during storage in ice or water with ice. Bull Tokai Reg Fish Res Lab 104:67–75 (in Japanese with English abstract)
Acknowledgements
This study was supported in part by a Grant-in-Aid (Grant Number 16K00822 and 25350100) for Scientific Research from the Japan Society for the Promotion of Science.
Author information
Authors and Affiliations
Corresponding author
Electronic supplementary material
Below is the link to the electronic supplementary material.
Rights and permissions
About this article
Cite this article
Tanimoto, S., Kikutani, H., Kitabayashi, K. et al. Qualitative changes in each part of yellowtail Seriola quinqueradiata flesh during cold storage. Fish Sci 84, 135–148 (2018). https://doi.org/10.1007/s12562-017-1157-2
Received:
Accepted:
Published:
Issue Date:
DOI: https://doi.org/10.1007/s12562-017-1157-2