Abstract
Due to global warming, high temperature has become the main abiotic stress affecting plant growth worldwide. LysM-containing receptor-like kinase 4 (LYK4) is the receptor for chitin, and ERECTA(ER) is a key factor in plant tolerance to high temperature. In this study, we constructed a chitin-induced chimeric LYK4-ER gene, in which the extracellular region and transmembrane domain of the LYK4 gene are fused with the intracellular region of the ER gene. Colony PCR, RT-PCR and western blot analyses of LYK4-ER transcription in plants, confirmed that the LYK4-ER gene was successfully constructed and transferred into Arabidopsis. The LYK4-ER gene localized to the cytomembrane and cytoplasm in vivo because of the binding properties of the transmembrane domain of the LYK4-ER gene to the cell membrane. The transgenic plants showed a higher germination rate and germination index as well as a shorter mean germination time than the wild-type plants, indicating that the LYK4-ER gene increases the heat tolerance of Arabidopsis. The lower H2O2 content and relative electrolytic leakage of the transgenic plants showed that the status of these plants under heat stress was improved. UPLC-MS/MS was used to analyze the phytohormones content, which suggested that the transgenic plants exhibited improved heat tolerance through jasmonic acid signal transduction pathways.
Similar content being viewed by others
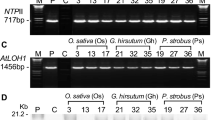
Avoid common mistakes on your manuscript.
Introduction
Rapid climate changes have occurred in recent centuries, with a large increase in CO2 concentration from approximately 270 μmol/mol in 1750 to more than 385 μmol/mol at present (Solomon 2007). As an inevitable consequence, higher temperatures have become the major abiotic stress affecting the plant growth, development and productivity worldwide. Heat normally reduces photosynthetic activity and the water content within the fresh weight by negatively affecting the cell growth and division. For example, the rice grain yield declines by 10% for each 1 °C increase in the local night temperature in the dry season (Peng et al. 2004). In response to the heat stress, the major events of plants are the perception and transduction of stress signals by signaling components, leading to the activation of stress-related genes. Numbers functional genes that respond to heat stress have been studied (Sonna et al. 2002; Zhang et al. 2005; Guo et al. 2016). For example, the best-known gene family responding to heat stress is the plant heat shock factors (HSFs), a thermotolerance can be enhanced by overexpressing Arabidopsis HSFA2, rice HSFA2e and lily HSFA2 in Arabidopsis (Guo et al. 2016). The ER gene controls the plant transpiration efficiency by affecting stomatal density, epidermal and mesophyll development and the porosity of leaves in Arabidopsis (Masle et al. 2005). Further studies have shown that the ER gene responds to heat stress not only in Arabidopsis (Qi and Hai 2004) but also in rice and tomato (Shen et al. 2015). Knockout or reduced expression of the ER allele decreases the thermotolerance of plants; thus, transgenic lines overexpressing ER show increased heat tolerance.
Plant priming can contribute to the response to an environmental stress and act as a cue to cope with the stress factor. Following the perception of the priming signal in the plant priming state, the activation of the protective responses is faster and stronger as long as the pressure exerted by the stress is accounted for. Chemical priming has become a promising strategy in fields related to plant stress physiology and crop stress management. Plants pretreated with chemical agents show enhanced heat tolerance compared to controls (Li et al. 2013; Anastasis et al. 2014; Sagor et al. 2013). For example, pretreatment with the nitric oxide donor sodium nitroprusside improves the survival percentage of maize seedlings and alleviats the increase in electrolyte leakage and the decrease in tissue vitality as well as the accumulation of malondialdehyde, indicating the promotion of the heat tolerance of maize seedlings (Li et al. 2013). Guan reported that seed priming via treatment with chitosan improved the speed of germination under chilling stress (Guan et al. 2009).
Chitin, a linear polymer of β-1,4-linked N-acetyl-glucosamine units, is the unique natural alkaline polysaccharide and a substantial component of fungal cell walls. Chitosan is generally produced via the deacetylation of chitin with the degree of deacetylation (%DD) in the ranging from 55 to 100%. Chitosan oligomers (COS), hydrolyzed from chitosan, exhibit better water solubility. COS have been characterized and shown act as a pathogen-associated molecular pattern that induces plant immunity against fungal and bacterial infection (Boller 1995; Ramonell et al. 2005; Shibuya and Minami 2001). Previous studies have indicated that lysin motif (LysM)-containing proteins are necessary for the plant recognition of chitin. In Arabidopsis, chitin elicitor receptor kinase 1 (CERK1)/LysM-containing receptor-like kinase 1(LYK1) responds to chitin-induced signaling. COS with a degree of polymerization greater than 5 promoted the dimerization of CERK1 and activate intracellular kinase domain phosphorylation, leading to plant immune responses (Miya et al. 2007; Petutschnig et al. 2010; Liu et al. 2012). There are five LYK genes in Arabidopsis: CERK1, LYK2, LYK3, LYK4 and LYK5. However, LYK2 or LYK3 does not show a significant effect on the recognition of chitin or active chitin-induced genes. A recent study showed that LYK4 participates in the chitin recognition and transduction, contributing to chitin signaling in plant innate immunity. lyk4 mutants show reduced induction of chitin-responsive genes, decreased chitin-induced cytosolic calcium elevation, and increased susceptibility to both the bacterial pathogen and fungal pathogen (Wan et al. 2012).
To the best of our knowledge, few studies have shown that signaling molecules exhibit specific binding to abiotic stress in plants. Therefore, constructing chimeric receptors with specific binding sites using in vitro DNA technology could be an effective way to enhance the thermotolerance of plants. Moreover, using chemical agents as signal molecules could further increase plant heat tolerance through chemical priming pathways. In this study, chitosan was selected as the signal molecule of interest due to its biodegradability, biocompatibility, antimicrobial activity, nontoxicity, and potential to enhance plant thermotolerance via chemical priming. We connected the extracellular region and transmembrane domain of the LYK4 gene with the intracellular region of the ER gene by overlap PCR to construct a chitin-induced LYK4-ER chimeric receptor. The chitin signal was recognized by the specific binding site of LYK4 protein and then transferred into the cell via the transmembrane domain of LYK4 protein, ultimately activating the intracellular domain of ER gene, improving the heat tolerance of the plant.
Materials and Methods
Construction of LYK4-ER Plasmids
The coding sequences of the extracellular region and transmembrane domain of LYK4 (1–300 bp) and the intracellular region of ER (600–979 bp, without a stop codon) were obtained from full-length sequences of LYK4 and ER, respectively, before the PCR-amplified overlap step. The PCR fragments were ligated into the plasmid provided by our lab, between the 35S promoter and the green fluorescence protein (GFP) or 6FLAG tag sequence. The primers used for overlap PCR (1–4) are shown in Table 1.
Identification of LYK4-ER Fusion Gene
Colony PCR analysis and Western blot analysis were used to identify the LYK4-ER-GFP (C4) and LYK4-ER-6FLAG (F4) genes in Escherichia coli (DH5α) or Agrobacterium tumefaciens (GV3101). PCR analysis was performed with primers 1 and 4 based on the template of DH5α or Agrobacterium tumefaciens GV3101 carrying the fusion gene. The protein was extracted from Arabidopsis thaliana via the liquid nitrogen grinding method after Agrobacterium-mediated infection. Then, the protein was identified by Western blot analysis.
RNA Extractions and Quantitative RT-PCR
The seeds of wild-type, transgenic LYK4-ER-GFP and LYK4-ER-Flag seedlings were used to extract total RNA using TRIzol Reagent (Invitrogen) according to the manufacturer’s protocol. After DNAse I treatment, first-strand cDNA was synthesized using 2 μg of total RNA in a 20 μL volume using M-MuLV reverse transcriptase (Fermentas) with an oligo(dT) 18 primer. The relative transcript level of each gene was determined by real-time PCR using SYBR green I master mix and a LightCycler 96 PCR machine (Roche), as described previously. At least three biological replicates were used to confirm the gene expression patterns, and PP2 was used as an internal gene expression control. The primers used in RT-PCR (5–8) are shown in Table 1.
Subcellular Localization of LYK4-ER-GFP
To observe the subcellular localization of the LYK4-ER protein, the plasmid with the fusion gene LYK4-ER-GFP was amplified with primers 1 and 4 (as shown in Table 1) from DH5α before being transferred into GV3101 for plant transformation. Then, the plasmid was transformed into tobacco leaf cells via Agrobacterium-mediated infection. Following pre-incubation at 28 °C for 48 h, the GFP signal was observed with a fluorescence microscope.
Plant Transformation and Growth Conditions
Arabidopsis thaliana carrying the chimeric LYK4-ER gene was generated by Agrobacterium tumefaciens-mediated transformation. The transformed lines were selected using kanamycin and identified by western blotting to check fusion gene expression. The A. thaliana Columbia ecotype was used as a control in these experiments. Plants were grown in black soil and vermiculite (1:3) in pots or on agar Petri dishes at 22 °C after 3 days of vernalization at 4 °C. For kanamycin selection, seeds of the LYK4-ER-overexpressing transgenic plants were surface-sterilized and sown on 1/2 MS medium with 1% sucrose, 0.7% agar, and 50 mg/L kanamycin. The T2 plants that produced 100% kanamycin-resistant plants in the T3 generation were considered homozygous transformants and used for further experiments.
Hypocotyl Length of LYK4-ER Plants
Chitosan was added to 1/2 MS medium at a final concentration of 0.2, 2 or 20 mg/L. The seeds were soaked in deionized water in 1.5 ml tubes for 3 days at 4 °C and then sterilized with NaClO for 6 min. After washing 3 times with bacterium-free water, the seeds were uniformly distributed on 1/2 MS medium with various concentrations of chitosan and 1.0% agar in sterile Petri dishes, which were oriented vertically and incubated at 22 °C for 7 days. Hypocotyl length was measured with ImageJ.
Heat Stress Treatment and Chitosan Treatment
Chitosan solutions of 0.2%, 0.6% and 1.0% (w/v) were used for seed priming. The seeds were soaked in deionized water in 1.5 ml tubes for 2 days at 4 °C before they were transferred to chitosan solutions with different concentrations for 16 h at 4 °C. For heat stress treatment, the seeds in 1.5 ml tubes were heat treated in a 45 °C water bath for 4 h after removal from the cold conditions, and the seeds were then sterilized with NaClO for 6 min. After washing 3 times with bacterium-free water, the seeds were uniformly distributed on 1/2 MS medium with 1% sucrose and 0.7% agar in sterile Petri dishes at 22 °C for 7 days, and germination rates were measured every day.
Measurement of Germination
Seed germination experiments were carried out after heat stress and chitosan treatment. Approximately 50 seeds were used for each treatment. Seeds were incubated at 22 °C under 24 h illumination. A seed was considered to have germinated when the germ was longer than the seed. The number of germinated seeds was counted every day for 7 days. The GR, GI and MGT were considered as evaluation criteria. GI = ∑(Gt/Tt) and MGT = ∑(Gt × Tt)/∑Gt are the formulas for GI and MGT, respectively, where Gt is the percentage of germination rate on Day t, and Tt is time corresponding to Gt in days; percentage data were arcsine-transformed before analysis according to the equation y = arcsin√(x/100).
Determination of Hydrogen Peroxide Content After Heat Stress
Twenty-eight-day-old seedlings were sprayed with a 0.2 mg/mL chitosan solution at 22 °C, and 2 h later, the seedlings were transferred to a 45 °C incubator for 4 h. A quantitative H2O2 assay kit (Solarbio Science & Technology Co, China) was used to determine the H2O2 content after chitosan treatment and heat stress. The experimental procedures were conducted according to the kit instructions: an approximately 0.1 g plant sample was homogenized with 1 mL cold acetone in an ice bath, and the resulting supernatant liquid was subjected to measurement after high-speed centrifugation.
Determination of Relative Electrolytic Leakage After Heat Stress
The procedures for chitosan and heat stress treatment were the same as in the quantitative H2O2 analysis. After heat stress treatment, 0.1 g plant material was soaked in 10 mL ddH2O. The tubes were placed in a vacuum dryer for 5 min and allowed to stand for 20 min before measuring electrolytic leakage (Ta). Then, the tubes were heated to 100 °C for 10 min, and electrolytic leakage was measured in the same way (Tb). The rate of relative electrolytic leakage = (Ta/Tb) × 100%.
Phytohormone Extraction and Analysis
The procedures for chitosan and heat stress treatment were the same as in the quantitative H2O2 analysis. After heat stress treatment, fresh plant tissue was frozen in liquid N2 and ground in a mortar. After being quickly weighed, 1 mL of 80% methanol with 0.1% formic acid was added to a 2 mL centrifuge tube, and the samples were blended and held at 4 °C overnight. Then, the extract samples were centrifuged for 10 min at 4 °C at 8000 rpm. The supernatant was transferred to a new tube, and the sediment was re-extracted with 1 mL of extraction solution. The supernatants were applied to and concentrated in a pressure blowing concentrator, and the extracts were then applied to a Waters Sep-pak C18 cartridge. The cartridge was washed with 2 mL of 0.1% formic acid solution and eluted with 2 mL of methanol. Finally, the eluted solution was dried and redissolved with 300 μL of methanol for UPLC-MS/MS (Waters Co, United States) analysis. The contents of abscisic acid (ABA), JA and salicylic acid (SA) were measured in this experiment.
Results
Construction and Identification of LYK4-ER Plasmids and Transgenic Plants
Figure 1 shows the construction of the fusion gene. The extracellular region and transmembrane domain of LYK4 (1–300 bp) and the intracellular region of ER (600–979 bp, without stop codon) were inserted between the 35S promoter and the GFP or 6FLAG tag sequence. Two LysM domains of the LYK4 gene (the key domain for chitosan signal recognition) were present in the fusion gene. Hence, the COS signal would be transferred to the response domain of the ER gene via the transmembrane domain of the LYK4 gene. Then, we transformed the newly constructed plasmid into DH5α, and colony PCR analysis was performed to identify the fusion gene. As shown in Fig. S1 (A), a single band of approximately 2 kb was present in DH5α, and the same result was observed in GV3101. Quantitative RT-PCR results also showed a high transcriptional level of LYK4-ER in the transgenic LYK4-ER-GFP or LYK4-ER-Flag seedlings in comparison with that in wild-type lines (Fig. 1b, c). A 105-kD band and a 98-kD band were observed in LYK4-ER-GFP and LYK4-ER-6FLAG transgenic plants (Fig. S1 B&C), which was consistent with the predicted molecular mass, indicating that the LYK4-ER-GFP and LYK4-ER-6FLAG fusion genes were successfully translated in Arabidopsis.
Schematic representation and quantitative RT-PCR of chimeric receptor constructs. (a) The transmembrane domain was marked in blue and pink rectangles were used for distinguishing different structural domains. (b) (c) Quantitative RT-PCR analysis of the transcriptional level of LYK4-ER in three transgenic LYK4-ER-GFP and LYK4-ER-6FLAG lines
Subcellular Localization of LYK4-ER-GFP Gene
The subcellular localization of the LYK4-ER protein was determined by the transient expression of the LYK4-ER-GFP fusion protein in tobacco leaf cells. GFP fluorescence was observed in both the cell membrane and cytoplasm (Fig. 2), indicating that LYK4-ER was localized to the cytomembrane and cytoplasm in vivo, similar to the LYK4 gene (Narusaka et al. 2013). Due to the integration of the transmembrane domain of LYK4-ER with the cell membrane, the fusion gene may have been anchored to the cell membrane. The localization of the LYK4-ER gene to the cell membrane was the precondition for recognizing the COS signal and transferring the signal into the cell via the extracellular region and transmembrane domain of the LYK4 protein, ultimately activating the intracellular domain of the ER gene to increase the heat tolerance of plants.
Hypocotyl Length of LYK4-ER Plants
In 7-day-old seedlings, we observed that the length of the hypocotyl in the transgenic lines was significantly increased compared to that of the wild-type seedlings (Fig. 3). This result was consistent with the findings of Qu et al. (2017). The ER gene regulates hypocotyl length by controlling cell elongation in Arabidopsis. Overexpressing the ER gene increased the hypocotyl length, while the ER family mutants showed a reduced plant height. The most pronounced effect of chitosan was recorded at a 20 mg/L concentration, and the hypocotyl length of both lines reached 3.9 mm.
Seed Germination Under Heat Stress
Figure 4a shows the germination phenotype of seeds after COS treatment and heat stress. The seeds of the wild-type plants and transgenic plants were evenly distributed in three areas of the same Petri dish to ensure that the three kinds of seeds were in the same external environment. The difference between the wild-type plants and the transgenic plants was visible to the naked eye, and the GR of the wild-type plants was obviously lower than that of the transgenic plants. Figure 4b, c show the control groups, in which seeds were germinated without heat stress after treatment with or without COS. Whether treated with COS or not, the transgenic plants showed a higher GR on the second day, but the final GR of each group was over 98%, indicating that the seeds were in a good state. As shown in Fig. 4d, e, GR and GI showed significant increases for all samples compared to the wild-type plants after heat treatment. The GI of the wild-type plants was 0.23, which was at least 25% lower than that of the transgenic plants.
Seed germination of transgenic plants under heat stress. (a) Seed germination after 2 mg/ml COS treatment and heat stress, picture was taken on the 7th day; (b) Seed germination under 22 ℃ without COS treatment, control a; (c) Seed germination under 22 ℃ after 2 mg/ml COS treatment, control b; (d) Germination rate, (e) germination index and (f) mean germination time of seeds treated with various concentration of COS after heat stress. Each experiment was repeated at least three times
MGT is used to represent the sprouting rate of the seeds under heat stress (Fig. 4f). Similar to GR and GI, there was a significant difference between the transgenic plants and the wild-type plants for this parameter. The MGT of the transgenic plants was almost 1 day faster than that of the wild-type plants. Although the best COS concentration varied among the different lines, MGT showed a decreasing tendency after treatment with chitosan compared with ddH2O because the 35S promoter increased the expression level of the LYK4-ER gene, and chitosan only showed a limited effect. The results indicated that the LYK4-ER gene was activated by chitosan and promoted the heat tolerance of Arabidopsis at the seedling stage.
Determination of Hydrogen Peroxide Content and Relative Electrolytic Leakage After Heat Stress
As shown in Fig. 5, after chitosan treatment and heat stress, the wild-type 28-day-old seedlings showed a higher level of H2O2, with a concentration of 1.07 nmol/g FW, while the concentration in the transgenic plants was 0.76 nmol/g FW, indicating that the ROS balance of the wild-type plants was damaged more severely after heat stress. A similar result was shown for the rate of relative electrolytic leakage, where the level in the transgenic plants was 23.6% lower than that in the wild-type plants, revealing that the transgenic plants showed less cell membrane rupture under heat stress. Both results showed that the transgenic plants exhibited better heat tolerance after chitosan treatment.
H2 O2 content and rate of electrolytic leakage after heat stress. 28-days old seedlings were sprayed with 0.2 mg/mL chitosan solution at 22 ℃, and 2 h later, seedlings were transferred to 45 ℃ incubator for 4 h. H2 O2 content was measured with a H2 O2 Quantitative Assay Kit. Electrolytic leakage was measured after 5 mins’ vacuuming and 20 mins’ standing. Each experiment was repeated at least three times
Determination of ABA, JA and SA Content After Heat Stress
To determine the signal transduction pathways that played a role in the plants under heat stress, we measured the phytohormones of the wild-type plants and transgenic plants before and after heat stress. Figure 6a–c are UPLC-MS/MS chromatograms for standard samples of ABA, JA and SA, respectively, with a concentration of 1000 ng/mL, and all the peak shapes have a good appearance. As shown in Fig. 6d, the levels of ABA and JA were extremely low in transgenic plants, while the levels of SA were around 8 ng/g FW, which did not differ from those in the wild-type plants before heat treatment. After heat treatment, both the wild-type plants and transgenic plants showed higher ABA and JA levels, while the levels of SA did not change greatly, which suggests that the heat stress signal was mainly transmitted through the ABA and JA signal transduction pathways. In addition, the levels of JA in the transgenic plants were typically higher than those of the wild-type plants, indicating that the transgenic plants may achieve better heat tolerance through JA signal transduction pathways.
Analysis of phytohormones in Arabidopsis thaliana wild type and transgenic plants before and after heat stress. (a-c) UPLC-MS/MS chromatogram for stand sample of ABA, JA and SA with a concentration of 1000 ng/mL. (d) Levels of phytohormones before and after heat stress. 28-days old seedlings were sprayed with 0.2 mg/mL chitosan solution at 22 ℃, and 2 h later, seedlings were transferred to 45 ℃ incubator for 4 h. Plant tissues were extracted with extraction solution (80% methanol with 0.1% formic acid) at 4 ℃ for 12 h twice. After purifying with a SPE cartridge, samples were analyzed with UPLC-MS/MS. Each experiment was repeated at least three times
Discussion
There are few reports on abiotic stress stimulated using defense mechanism inducers with good results (Guan et al. 2009; Ruan and Xue 2002) because most of the related studies are focused on generating crops with intrinsic resistance to abiotic stress based on traditional breeding techniques. In our research, we constructed a fusion gene with specific binding sites for chitosan, which makes the heat stress signal easier to recognize and control by chemical priming. Then, the intracellular region of the ER gene was activated when the signal was recognized and transported by the LYK4 gene, leading to the improved heat tolerance of Arabidopsis at the seedling stage.
There are many factors associated with the effects of seed priming used to control the hydration level within seeds, in which the concentrations of the priming solutions and the time and temperature of priming are crucial (Adegbuyi et al. 1981; Khan 1992). Previous research showed that chitosan forms a protective coating on maize seeds under biotic stresses that allows seedlings to develop defense mechanisms against the fungi A. flavus and F. moniliforme, which manifests in a decrease or absence of disease in different structures of the seedlings (Lizárraga-Paulín et al. 2011). In the present study, we found that the combination of the mean germination time and concentration differed between the wild-type and transgenic plants. After treatment with chitosan, the wild-type only showed an increased GR, but no significant differences were found in GI and MGT, indicating that chitosan had the potential to increase the plant germination rate under heat stress via chemical priming. In the transgenic plants, because of the special binding site and transmembrane domain of the LYK4 gene, the chitosan signal could be transformed into the cell more effectively, leading to a more evident difference between the plants with or without chitosan priming, and a higher germination index and sprout speed were observed in the chitosan-treated lines.
Plant aerobic metabolic processes, such as respiration and photosynthesis, continuously produce reactive oxygen species (ROS) including singlet oxygen, superoxide, hydroxyl radicals and hydrogen peroxide (H2O2) as byproducts. Under stress-free conditions, the balance of ROS is maintained under the control of plant secondary metabolites such as superoxide dismutase (SOD), catalase (CAT), and ascorbate peroxidase (APX). This balance is destroyed when the plant is subjected to heat stress, leading to an increased content of H2O2 (Khedia et al. 2019), mainly due to the change in oxidoreductase activity, in addition to the enhancement of the Mehler reaction. In this study, the transgenic plants showed lower levels of H2O2 than the wild-type plants under heat stress, suggesting that the chimeric LYK4-ER gene could protect the photosynthetic apparatus from oxidative stress induced by high-temperature stress.
Plant hormones are structurally diverse compounds that are grouped into several major classes (i.e., auxins, cytokinins, ABA, gibberellins, ethylene, JA, SA and brassinosteroids). Among these hormones, ABA, JA and SA are known to play pivotal roles in plant growth, development and responses to biotic and abiotic stress (Davies 2004; Kanchan et al. 2017). ABA is known to stimulate short-term responses such as the closure of stomata, resulting in the maintenance of water balance (Zhang et al. 1987) and longer-term growth responses through the regulation of stress-responsive genes under drought stress. SA and JA are mainly known to play significant roles in activating plant defense mechanisms in response to pathogen attack (Bari and Jones 2009). In the present study, chitosan treatment and heat stress increased the contents of ABA and, especially, JA simultaneously. These findings were consistent with Baron’s (Baron et al. 2012) and Manvi’s (Manvi and Ashverya 2016) studies, but it is still unclear how the three phytohormones work together.
Plants harboring loss-of-function alleles of ER were more sensitive to heat stress than wild-type plants and restored heat tolerance was observed after transforming the ER gene and its native promoter into the null mutant. However, the mechanisms whereby the overexpression of ER confers thermotolerance have not yet been defined in Arabidopsis or other organisms. Although ER positively regulates transpiration efficiency, no difference in water content is observed between the null er mutant and the wild type (Shen et al. 2015). In summary, considering that the ER gene can respond to heat stress not only in Arabidopsis but also in rice and tomato, genetic and biochemical approaches may be very useful tools for addressing these developmental questions in economic crops.
Conclusions
A chitin-induced chimeric LYK4-ER gene was successfully constructed, and the fusion gene improved the germination rate, germination index and sprout speed of Arabidopsis after heat stress. Chitosan treatment increased the germination rate of the wild-type plants and further reduced the mean germination time of the transgenic plants. The transgenic plants showed a lower H2O2 content and relative electrolytic leakage, indicating that the transgenic plants remained in better condition than the wild-type plants under heat stress. The transgenic plants may achieve better heat tolerance via JA signal transduction pathways. In general, chimeric genes show high potential for developing the heat tolerance of Arabidopsis and other plants.
References
Adegbuyi E, Cooper SR, Don R (1981) Osmotic priming of some herbage grass seed using polyethylene glycol (PEG). Seed Sci Technol 9(3):867–878
Anastasis C, Panagiota F, Manganaris GA et al (2014) Sodium hydrosulfide induces systemic thermotolerance to strawberry plants through transcriptional regulation of heat shock proteins and aquaporin. BMC Plant Biol 14(1):42–42
Bari R, Jones JDG (2009) Role of plant hormones in plant defence responses. Plant Mol Biol 69(4):473–488
Baron KN, Schroeder DF, Stasolla C (2012) Transcriptional response of abscisic acid (aba) metabolism and transport to cold and heat stress applied at the reproductive stage of development in Arabidopsis thaliana. Plant Sci 188–189:0–59
Boller T (1995) Chemoperception of microbial signals in plant cells. Annu Rev Plant Physiol Plant Mol Biol 46(1):189–214
Davies PJ (2004) Plant hormones: biosynthesis, signal transduction, action [M]. Kluwar Academic Publishers, Norwell, pp 1–15
Guan YJ, Hu J, Wang XJ et al (2009) Seed priming with chitosan improves maize germination and seedling growth in relation to physiological changes under low temperature stress. J Zhejiang Univ Sci B (Biomedicine & Biotechnology) 10(6):427–433
Guo M, Liu JH, Ma X et al (2016) The Plant heat stress transcription factors (HSFs): structure, regulation, and function in response to abiotic stresses. Front Plant Sci 7(273):114
Kanchan V, Neha U, Nitin K et al (2017) Abscisic acid signaling and abiotic stress tolerance in plants: a review on current knowledge and future prospects. Front Plant Sci 08:1–12
Khan AA (1992) Preplant physiological seed conditioning. Hortic Rev 13:131–181
Khedia J, Agarwal P, Agarwal PK (2019) Deciphering hydrogen peroxide-induced signalling towards stress tolerance in plants. Biotech 9(11):395
Li ZG, Yang SZ, Long WB et al (2013) Hydrogen sulphide may be a novel downstream signal molecule in nitric oxide-induced heat tolerance of maize (Zea mays L.) seedlings. Plant Cell Environ 36(8):1564–1572
Liu T, Liu Z, Song C et al (2012) Chitin-induced dimerization activates a plant immune receptor. Science 336(6085):1160–1164
Lizárraga-Paulín EG, Torres-Pacheco I, Moreno-Martínez E et al (2011) Chitosan application in Maize (Zea mays) to counteract the effects of abiotic stress at seedling level. Afr J Biotech 10(34):6439–6446
Manvi S, Ashverya L (2016) Jasmonates: emerging players in controlling temperature stress tolerance. Front Plant Sci 6:1–10
Masle J, Gilmore SR, Farquhar GD (2005) The ERECTA gene regulates plant transpiration efficiency in Arabidopsis. Nature 436(7052):866–870
Miya A, Albert P, Shinya T et al (2007) CERK1, a LysM receptor kinase, is essential for chitin elicitor signaling in Arabidopsis. Proc Natl Acad Sci USA 104(49):19613–19618
Narusaka Y, Shinya T, Narusaka M et al (2013) Presence of LYM2 dependent but CERK1 independent disease resistance in Arabidopsis. Plant Signal Behav 8(9):e25345
Peng S, Huang J, Sheehy JE et al (2004) Rice yields decline with higher night temperature from global warming. Proc Natl Acad Sci USA 101(27):9971–9975
Petutschnig EK, Jones AME, Serazetdinova L et al (2010) The lysin motif receptor-like kinase (LysM-RLK) CERK1 is a major chitin-binding protein in Arabidopsis thaliana and subject to chitin-induced phosphorylation. J Biol Chem 285(37):28902–28911
Qi Y, Hai H (2004) ERECTA is required for protection against heat-stress in the AS1/AS2 pathway to regulate adaxial-abaxial leaf polarity in Arabidopsis. Planta 219(2):270
Qu XY, Zhao Z, Tian ZX (2017) ERECTA regulates cell elongation by activating auxin biosynthesis in Arabidopsis thaliana. Front Plant Sci 8:1688
Ramonell K, Berrocal-Lobo M, Koh S et al (2005) Loss-of-function mutations in chitin responsive genes show increased susceptibility to the powdery mildew pathogen Erysiphe cichoracearum. Plant Physiol 138(2):1027
Ruan SL, Xue QZ (2002) Effects of chitosan coating on seed germination and salt-tolerance of seedlings in hybrid rice (Oryza sativa L.). Acta Agronom Sin 28(6):803–808
Sagor GH, Berberich T, Takahashi Y et al (2013) The polyamine spermine protects Arabidopsis from heat stress-induced damage by increasing expression of heat shock-related genes. Transgen Res 22(3):595
Shen H, Zhong X, Zhao F et al (2015) Overexpression of receptor-like kinase ERECTA improves thermotolerance in rice and tomato. Nat Biotechnol 33(9):996
Shibuya N, Minami E (2001) Oligosaccharide signalling for defence responses in plant [J]. Physiol Mol Plant Pathol 59(5):223–233
Solomon S (2007) Contribution of working group I to the fourth assessment report of the intergovernmental panel on climate change, climate change 2007: the physical science basis. Cambridge University Press, New York, pp 159–254
Sonna LA, Fujita J, Gaffin SL et al (2002) Invited review: effects of heat and cold stress on mammalian gene expression. J Appl Physiol 92(4):1725–1742
Wan J, Tanaka K, Zhang XC et al (2012) LYK4, a LysM receptor-like kinase, is important for chitin signaling and plant innate immunity in Arabidopsis. Plant Physiol 160(1):396–406
Zhang J, Schurr U, Davies et al (1987) Control of stomatal behaviour by abscisic acid which apparently originates in the roots. J Exp Bot 38(7):1174–1181
Zhang Y, Mian MA, Chekhovskiy K et al (2005) Differential gene expression in Festuca under heat stress conditions. J Exp Bot 56(413):897–907
Author information
Authors and Affiliations
Corresponding author
Electronic Supplementary Material
Below is the link to the electronic supplementary material.
Rights and permissions
About this article
Cite this article
Chen, L., Xia, W., Song, J. et al. The Chitin-Induced Chimeric LYK4-ER Gene Improves the Heat Tolerance of Arabidopsis at the Seedling Stage. J. Plant Biol. 63, 279–288 (2020). https://doi.org/10.1007/s12374-020-09249-3
Received:
Revised:
Accepted:
Published:
Issue Date:
DOI: https://doi.org/10.1007/s12374-020-09249-3