Abstract
Majority of animals form symbiotic relationships with bacteria. Based on the number of bacterial species associating with an animal, these symbiotic associations can be mono-specific, relatively simple (2–25 bacterial species/animal) or highly complex (>102–103 bacterial species/animal). Photorhabdus (family-Enterobacteriaceae) forms a mono-specific symbiotic relationship with the entomopathogenic nematode Heterorhabditis. This system provides a tractable genetic model for animal-microbe symbiosis studies. Here, we investigated the bacterial factors that may be responsible for governing host specificity between nematode and their symbiont bacteria using proteomics approach. Total protein profiles of P. luminescens ssp. laumondii (host nematode- H. bacteriophora) and P. luminescens ssp. akhurstii (host nematode- H. indica) were compared using 2-D gel electrophoresis, followed by identification of differentially expressed proteins by MALDI-TOF MS. Thirty-nine unique protein spots were identified - 24 from P. luminescens ssp. laumondii and 15 from P. luminescens ssp. akhurstii. These included proteins that might be involved in determining host specificity directly (for e.g. pilin FimA, outer membrane protein A), indirectly through effect on bacterial secondary metabolism (for e.g. malate dehydrogenase Mdh, Pyruvate formate-lyase PflA, flavo protein WrbA), or in a yet unknown manner (for e.g. hypothetical proteins, transcription regulators). Further functional validation is needed to establish the role of these bacterial proteins in nematode-host specificity.
Similar content being viewed by others
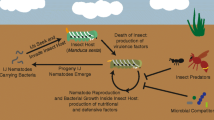
Avoid common mistakes on your manuscript.
Introduction
Animal-microbe relationships are a fact of life. These associations can be simple mono-specific associations (for e.g. nematodes-bacteria symbiosis), relatively simple consortia (2–25 bacterial species in an animal, for e.g. leach gut consortium, insect gut consortium) and highly complex consortia (for e.g. vertebrate guts colonized by >102–103 species of bacteria) [1]. Symbionts affect the physiology, immunity, metabolism, behaviour, growth and development of host, and offer protection to the host [2, 3]. It is astonishing that trillions of bacteria can live symbiotically in a vertebrate gut without eliciting any potentially harmful host immune response. The mechanisms which govern the host-symbiont association patterns are not very well known. The microbial complexity of the insect and vertebrate guts is a major limiting factor in understanding the microbe-animal interactions in these models.
Photorhabdus is a gram negative enterobacterium which is found in nature only in the gut of the nematodes of the genus Heterorhabditis [4]. Photorhabdus are known to produce an array of toxins and secondary metabolites [5, 6], and together with their nematode-hosts they are potent insect killers. The Photorhabdus–Heterorhabditis pair provides a tractable genetic model to study animal-microbes relationships [7]. These nematodes co-evolved with their bacterial symbionts for millions of years; and form very specific symbiotic relationships and mostly are not colonized by other strains and species of Photorhabdus [8]. Through many cross-colonization experiments performed in the laboratories, it has been established that Photorhabdus and Heterorhabditis symbiotic relationships are highly specific [8]. Since Heterorhabditis nematodes are used for the biological control of insects in agricultural crops, this bacteria-nematode specificity is an important factor for fermenter based commercial mass production of nematodes as the nematodes can multiply and develop only on their specific symbiont Photorhabdus strain [9, 10]. The genomes of the bacterial symbiont P. luminescens ssp. laumondii and its nematode-host H. bacteriophora TT01 have been sequenced [4, 11], and are available in public domain. Recently, the details of symbiont colonization sites in the nematode gut, and the developmental progression of this symbiotic relationship has been elucidated for Photorhabdus–Heterorhabditis pair [12]. Some of the bacterial genes and processes necessary for symbiotic colonization of the nematodes have also been identified [13–16].
Comparative genomics has been used to understand genomic diversification for niche expansion, for e.g. in Lactobacillus [17]. Similarly, genomics based approaches led to significant advancements in understanding the mechanisms involved in Photorhabdus–Heterorhabditis symbiosis, but our knowledge about the factors that govern host specificity per-se is rather limited. In the symbiosis between nematode Steinernema carpocapsae and its symbiont Xenorhabdus nematophila, it was found that X. nematophila nilABC, a single genetic locus encoding for membrane proteins was necessary and sufficient for determining initial colonization specificity [18]. The only study to determine genomic regions involved in host specificity in Photorhabdus–Heterorhabditis system by comparing gDNA of P. luminescens ssp laumondii TT01 with P. temparata XlNach strains in a microarray hybridization experiment identified 8 genomic regions possibly involved in host specificity [19]. However, lack of functional validation to support the findings in this study warranted further investigations.
The developments in the field of quantitative proteomics have revolutionized the field of biological research [20]. In case of insect parasitic nematodes and their symbiont bacteria, proteomics based global approaches were used in investigation of desiccation stress tolerance in entomopathogenic nematodes Steinernema [21], and for the analysis of phase variation process in the bacterium Photorhabdus [16]. We hypothesize that the host specificity may be caused by the differences in genetic constitution of the symbiont bacterial species, which might be reflected in their respective proteomes. Here, we used 2D-gel based approach to compare proteomes of two Photorhabdus species that do not colonize each other’s nematode-host to identify the proteins that may govern host specificity between Heterorhabditis and Photorhabdus.
Materials and Methods
Strains and Culture Conditions
The nematode H. indica was isolated from Nagpur, Maharashtra, India (ITS accession No. HQ637414), and the nematode H. bacteriophora TTO1 was a gift from Dr. Byron Adams, BYU, USA to Dr. Sudershan Ganguly. Isolation of bacterial symbionts was done from the nematode infective juveniles (IJs). Freshly harvested IJs from White’s trap were collected and surface sterilized by using 2 % commercial bleach and sterilized double distilled water. The surface sterilized IJs were manually crushed by tissue grinder, and one loop of this suspension was streaked on Petri-plates containing nutrient bromothymol blue agar (NBTA, in 1 litre water: 3 g beef extract, 5 g peptone, 0.025 g bromothymol blue and 0.04 g 2,3,5- triphenyl tetrazolium chloride, 15 g Agar, pH 7.2) and incubated at 28 °C for 48 h. Pure green colonies were isolated and confirmed as Photorhabdus by sequencing of 16s rDNA. The symbiont bacteria isolated from H. indica was identified as P. luminescens ssp. akhurstii (GenBank accession no. JX240394), and from H. bacteriophora as P. luminescens ssp. laumondii, and were used for isolation of total protein.
Isolation of Total Protein from Bacteria
The bacteria were cultured in Luria broth (LB) in an incubator shaker (28 °C, 48 h at 200 rpm). The cultures were centrifuged (6000 rpm, 8 °C) to harvest the cells. 10 mM sodium phosphate buffer (pH 6.0) was used to wash the bacterial cells and then the cells were mechanically lysed by grinding in Tris-HCl buffer (50 mM, pH 7.0) in a 1.5 ml tube. The cellular debris was removed by centrifuging the lysed cells and filtering the supernatant through 0.2 µm membrane (Millipore, India). The protein was quantitated using the Bradford method.
2-D Gel Electrophoresis and Staining
Cleaning of extracted proteins was done by 2-D Clean-Up kit (Amersham Biosciences, Belgium). The protein pellet was rehydrated (rehydration buffer composition- 2 M Thiourea, 0.5 % Bioampholytes, 20 mM dithiothreitol, 7 M Urea). One hundred μg of purified protein was loaded on 11 cm IPG strips (GE Healthcare, USA) of pH 3–10 and 4–7. The first dimension separation of proteins was done on IEF cell (GE Healthcare, USA) at 200 V for 3 h. After this a gradient was applied for 2 h at 500 V, followed by final focusing at 8000 V for 2.5 h. The second dimension separation of proteins was done by using SDS-polyacrylamide gel (12.5 %), followed by silver staining. After the completion of electrophoresis run, the gels were incubated in a fixative solution (acetic acid-12 %, methanol-50 %), kept on rocking shaker at room temperature for 1 h, washed by 50 % ethanol followed by 30 % ethanol for 30 m, sensitized by 0.002 % sodium thiosulphate solution for 60 s, and then washed thrice for 20 s with distilled water. Silver staining of gel was done in 0.028 % formaldehyde and 12.0 mM AgNO3 solution for 20 m, and the gel was washed thrice by distilled water prior to processing in the developing solution (sodium carbonate- 6 %, formaldehyde- 0.0185 % and sodium thiosulphate- 4 %). Lastly, the gel was scanned at 300 dpi resolution using a scanner (CanoScan 8400F, Canon, Germany) to acquire the image of gels for identification of host specific molecules. The unique proteins were identified, excised, and sent for MALDI-TOF/TOF analysis.
In–Gel Digestion and Protein Identification
The completely resolved unique spots were excised and dehydrated in a vacuum centrifuge prior to the enzymatic digestion. Rehydration of the gel slices was done in trypsin (10.0 ng/µl in ammonium bicarbonate (100 mM, pH 7.4). The tubes were incubated overnight at 37 °C to allow complete protein digestion; thereafter extracted peptides were vacuum dried. MALDI-TOF analysis was done by outsourcing to Sandor Life Sciences Pvt.Ltd., Hyderabad, India. In brief, 1.0 µl of the peptide sample (reconstituted) was added into 2 consecutive columns (C18-reversed phase chromatography, and C18 PepMap nano-analytical column (LC Packings, Germering, Germany)). MALDI-TOF with a positive ion mode nano-flow ESI Z-spray source was utilized for analyzing chromatographically separated peptides. The data was captured by MassLynx software (v 4.0), processed by ProteinLynx Global Server (v 2.2, Micromass, Manchester, U.K.) as PKL (peak list) at standard parameters. Peak lists of peptides were searched against MASCOT (http://www.matrixscience.com) and NCBI nr protein database at standard parameters. The protein was called as ‘identified’ when the threshold P < 0.05 was exceeded by peptide ion score for 2 or more peptides, and the molecular mass and pI values matched in the corresponding gel(s). The experiment was replicated thrice.
Results
Total protein profiles of two subspecies of Photorhabdus bacteria that do not colonize each other’s nematode-host, i.e., P. luminescens ssp. laumondii (host- H. bacteriophora), and P. luminescens ssp. akhurstii (host- H. indica) were matched. Distinct differences in SDS-PAGE protein profiles were observed between 15–55 kDa molecular weight range suggesting proteomic divergence (Fig. 1). The differences in proteomes were further resolved using 2D-gel electrophoresis followed by sequencing of proteins unique to each bacterial subspecies. Isoelectric focusing of proteins at pH 3 to 10 revealed 4 unique spots in P. luminescens ssp. akhurstii and 8 in P. luminescens ssp. laumondii, respectively (Fig. 2a, b). Resolving the protein spots by expanding the pH range from 4 to 7 identified 11 and 16 additional unique spots in P. luminescens ssp. akhurstii and P. luminescens ssp. laumondii, respectively (Fig. 2c, d). In total, 39 unique protein spots were identified; 15 unique to P. luminescens ssp. akhurstii and 24 unique to P. luminescens ssp. laumondii.
2D-gel electrophoresis map of proteins at isoelectric point pH units 3–10 a P. luminescens ssp. akhurstii and b P. luminescens ssp. laumondii and isoelectric point pH units 4–7 c P. luminescens ssp. akhurstii and d P. luminescens ssp. laumondii. Thin black arrows indicate unique protein spots; thick black arrows indicate size of protein marker
These spots were identified by peptide mass mapping, followed by sequence search against UniProt (Table S1). The proteins unique to P. luminescens ssp. akhurstii, (Spot numbers 1–4, 13–23, Fig. 2, Table S1) were identified as O-acetylhomoserine amino carboxypropyl transferase; catalase (KatE); peptidase T (PepT); ABC transporter ATP-binding protein (AfuC); iron-containing alcohol dehydrogenase; ABC transporter-like protein (YhbG); hypothetical protein highly similar to probable transcription regulator AraC; hypothetical protein similar to conjugative transfer ATPase of PFL_4706 family; DNA topoisomerase IV subunit A, ParC; hypothetical protein similar to YjeE of Escherichia coli; hypothetical protein similar to flavoprotein WrbA (Trp repressor binding protein); dihydrolipoamide dehydrogenase IpdA; radical SAM domain-containing protein PflA; pyridoxine 5′-phosphate synthase, PdxJ, and methylglyoxal synthase, MgsA.
Similarly, proteins those were unique to P. luminescens ssp. laumondii (spot numbers 5–12 and 24–39, Fig. 2, Table S1) comprised of 2 hypothetical proteins not similar to any know protein; molecular chaperone protein DnaK; elongation factor G FusA; outer membrane protein A (ompA); phosphate transporter subunit PstS; glutamate and aspartate transporter subunit GltI; hypothetical protein similar to non-ribosomal peptide synthetase modules and related proteins EntF; phosphate regulon sensor kinase PhoR; phospho carrier protein NPr, ptsO; hypothetical protein similar to YcjX of E. coli; TolB; L-isoaspartate carboxyl methyltransferase Pcm; enolase (2-phosphoglycerate dehydratase); sulfate transport system permease protein CysW; small subunit of the acetolactate synthase isozyme III (AHAS-III), IlvH; pilin FimA; malate dehydrogenase Mdh; 2,3-bisphosphoglycerate-dependent phosphoglycerate mutase GpmA; triosephosphate isomerase TpiA; iron ABC transporter substrate-binding protein; amino-acid acetyltransferase (N-acetylglutamate synthase) ArgA; aromatic amino acid amino-transferase, AspC and molybdenum-binding transcriptional repressor ModE.
In summary, a set of 39 proteins was identified, 15 unique to P. luminescens ssp. akhurstii and 24 unique to P. luminescens ssp. laumondii.
Discussion
To identify proteins involved in conferring nematode-host specificity, we compared the total protein profiles of P. luminescens ssp. laumondii (nematode-host- H. bacteriophora) and P. luminescens ssp. akhurstii (nematode-host- H. indica) by 2D-gel electrophoresis. Several proteins (Fig. 2, spots 4, 13, 14, 15, 16, 20, 24, 31) did not run at theoretical molecular weight (Table S1) resulting in an anomaly which is commonly known as ‘gel shift’. Many membrane proteins are covalently bound to carbohydrates or lipid moiety hence it is expected that their molecular weight would be higher than theoretical molecular weight. However, several other factors are known to be responsible for this anomaly. The first reason could be the binding of proteins to SDS leading to formation of SDS-complexes and resulting in modification and oligomerization of proteins [22]. Other reasons could be post translational modifications like glycosylation, ubiquitination or phosphorylation etc., presence of acidic amino acids in the protein [23], incomplete unfolding of proteins, or presence of proline-rich regions which lend rigidity to the protein backbone. Lower observed molecular weight of some proteins could be due to truncation of proteins as some proteins carry protease sensitive motifs and may get cleaved off.
Some of the differences in the protein profile of the two Photorhabdus strains can definitely be attributed to the taxonomic differences between the compared strains. Few proteins identified as being unique in P. luminescens ssp. akhurstii are housekeeping proteins (for e.g. LpdA, ParC and KatE) and homologues are present in P. luminescens ssp. laumondii TTO1 based on genome sequence. Also, some proteins found as unique in P. luminescens ssp. laumondii TTO1 are important and conserved proteins (i.e. DnaK, Mdh), and are likely to be present in P. luminescens ssp. akhurstii. Some other proteins identified in our study are required for secondary metabolite biosysnthesis, for e.g. pyruvate formate-lyase PflA, flavo protein wrbA, Pyridoxine 5′-phosphate synthase PdxJ. We found these proteins as unique to either of the bacterial strains in this study. It is unlikely that these proteins are directly involved in determination of host specificity. However, these genes might regulate bacterial secondary metabolism in post-exponential growth phase and production of symbiosis factors (for e.g. bioluminescence, an anthraquinone pigment (AQ) and an antibiotic 3-5-dihydroxy-4-isopropylstilbene), and might affect host specificity and symbiosis indirectly. For e.g., Plu4547 (mdh) was previously identified to be involved in symbiosis between Photorhabdus and Heterorhabditis [14]. mdh encodes for malate dehydrogenase which is a key tricarboxylic acid (TCA) cycle enzyme. It was observed that nematode growth and development was not supported by an mdh mutant, establishing the role for secondary metabolism in bacteria-nematode symbiotic interactions [14]. Similarly, an alarmone (p)ppGpp is needed for the symbiont bacteria P. luminescens to sustain nematode development and growth through its effect on secondary metabolism. Therefore, without an in-depth investigation on role of each one of these housekeeping, essential and biosynthetic pathway proteins, it would be premature to speculate on their role in host specificity and symbiosis.
A subset of the identified proteins could be directly involved in modulating nematode-bacterium host specificity. Three proteins, i.e. O-acetylhomoserine amino carboxypropyl transferase (Plu3517); hypothetical protein, similar to non-ribosomal peptide synthetase modules and related proteins EntF (Plu3130); and P pilus assembly protein, pilin FimA (Plu0418) are present in P. luminescens ssp. laumondii TTO1, which is the bacterial symbiont of H. bacteriophora, but not in P. temperata ssp. temperata XlNach (host nematode-H. megidis). These three genes are present on three of the Photorhabdus genomic islands (GI) [4, 19]. The EntF protein is required for synthesis of a high affinity siderophore enterobactin [24]. It is a widely known fact that siderophores are involved in pathogenicity and host specificity of plant and animal pathogenic bacteria. A unique siderophore molecule might help the bacterial symbiont in colonizing its nematode-host. However, it remains to be seen if the structure of the same siderophore molecule varies between different Photorhabdus strains and species, and if this variability could lead to host specificity. Similarly, the pilin protein FimA is important in context of host specificity as fimbriae are bacterial adhesive organelle and facilitate host-nematode recognition and adhesion of the symbiont bacteria to a yet unknown receptor in the nematode intestine. It is possible that during the course of evolution, the pilins have co-evolved along with this nematode receptor leading to a high degree of host specificity recognition. Previously, a novel mad fimbrial locus (Plu0261-Plu0270) was found to be involved in symbiosis between the nematode and the bacteria, which supports this hypothesis [15, 25].
Discovering Plu1775 (outer membrane protein A) as unique in one of the strains is a significant finding. In a previous study to determine the factors responsible for host specificity in the bacterium X. nematophila, it was discovered that nilABC genes suggested to encode for various components of bacterial membrane proteins that were absent in eight other Xenorhabdus species but were present only in X. nematophila, thus underlining their role in host colonization and host specificity. Transfer of X. nematophila nilABC genes into two species of Xenorhabdus that did not colonize S. carpocapsae (X. poinarii and X. bovienii) enabled these species to symbiotically colonize S. carposapsae [18]. Similarly, in the Gram-negative bacteria, protein OmpA constitutes the outer membranes [26]. OmpA has many functions, including adherence to host tissues in several pathogenic bacteria (summarized in [26]). Difference in OmpA hypervariable domains has been linked to host specificity between cattle and sheep isolates of Mannheimia (Pasteurella) haemolytica, M. glucosida, and P. trehalosi [26]. Therefore, it is possible that Photorhabdus OmpA might be important in establishing symbiosis and host specificity with their nematode-hosts.
In addition, we identified components of 5 different ABC transporters-afuC (Plu0810), yhbG (Plu4040), pstS (Plu0214) from P. luminescens ssp. akhurstii, gltI (Plu1307) and Plu2853 from P. luminescens ssp. laumondii. A group of ABC transporters, lsr locus, was identified as one of the regions common and specific to Photorhabdus bacteria that formed symbiotic relationships with H. bacteriophora in a previous study [19]. The lsr locus encodes for proteins suggested to be involved in quorum sensing [19]. Therefore, there is possibility that other ABC transporter systems are involved in symbiosis through similar mechanisms. For e.g., in a phosphate ABC transporter, PstS is a phosphate-binding component also suggested to be involved in two-component signal transduction [27] whereas role for yhbG is not clear. Function of other ABC transporters identified in this study, i.e., ferric (afuC, Plu2853), glutamate and aspartate (GltI) transport are relatively well defined. Signaling is extremely important in determining the outcomes of host-bacterium interactions.
Two unique putative transcriptional regulators- Plu2244 and Plu1964 from P. luminescens ssp. akhurstii, and one (Plu1474) from P. luminescens ssp. laumondii TTO1 were discovered in this study. Plu2244 is a hypothetical protein similar to E. coli transcription factor AraC. AraC is a transcriptional regulator of arabinose catabolism and transport genes and operons [28]. Plu1964 is similar to transcriptional repressor wrbA which has role in stress responses [29]. Plu1474 encodes for a protein similar to a molybdate-dependent transcriptional regulator ModE, which in E. coli acts as a molybdate concentration sensor, and regulates transcription of operons involved in molybdenum uptake and utilization [30]. Other interesting genes identified in our study were five novel hypothetical proteins, Plu2059, Plu2582, Plu4585, Plu2060, and Plu1085. It has been found in more than one case that a single gene could regulate the bacterial host range, and thus specificity. The transcriptional regulators and hypothetical proteins are always an interesting candidate for the functional validation because they could be involved in a biological phenomenon in a number of unpredictable ways.
In summary, we identified a pool of 39 bacterial proteins that were different between P. luminescens ssp. akhurstii and P. luminescens ssp. laumondii TTO1. It is likely that a subset of these proteins, for e.g. pilin FimA, OmpA, might actually be involved in regulating host specificity and symbiosis with their nematode-hosts, either directly, or indirectly via their effect on secondary metabolism. These proteins present interesting targets for functional genetic validation to confirm their role in governing nematode-bacterium host specificity and symbiosis.
References
Dethlefsen L, McFall-Ngai M, Relman DA (2007) An ecological and evolutionary perspective on human-microbe mutualism and disease. Nature 449:811–818. doi:10.1038/nature06245
Kamada N, Seo SU, Chen GY, Nunez G (2013) Role of the gut microbiota in immunity and inflammatory disease. Nat Rev Immunol 13:321–335. doi:10.1038/nri3430
Sharon G, Segal D, Ringo JM, Hefetz A, Zilber-Rosenberg I, Rosenberg E (2010) Commensal bacteria play a role in mating preference of Drosophila melanogaster. Proc Natl Acad Sci USA 107:20051–20056. doi:10.1073/pnas.1009906107
Duchaud E, Rusniok C, Frangeul L, Buchrieser C, Givaudan A, Taourit S, Bocs S, Boursaux-Eude C, Chandler M, Charles JF, Dassa E, Derose R, Derzelle S, Freyssinet G, Gaudriault S, Medigue C, Lanois A, Powell K, Siguier P, Vincent R, Wingate V, Zouine M, Glaser P, Boemare N, Danchin A, Kunst F (2003) The genome sequence of the entomopathogenic bacterium Photorhabdus luminescens. Nature Biotechnol 21:1307–1313. doi:10.1038/nbt886
Kushwah J, Somvanshi VS (2015) Photorhabdus: a microbial factory of insect-killing toxins. In: Kalia VC (ed) Microbial factories: biodiversity, biopolymers, bioactive molecules, vol 2. Springer India, New Delhi, pp 235–240. doi:10.1007/978-81-322-2595-9_15
Inman FL III, Holmes L (2012) Antibacterial screening of secreted compounds produced by the phase I variant of Photorhabdus luminescens. Ind J Microbiol 52:708–709. doi:10.1007/s12088-012-0307-6
Ciche T (2007) The biology and genome of Heterorhabditis bacteriophora. WormBook. doi:10.1895/wormbook.1.135.1
Waterfield NR, Ciche T, Clarke D (2009) Photorhabdus and a host of hosts. Annu Rev Microbiol 63:557–574. doi:10.1146/annurev.micro.091208.073507
Inman FL, Singh S, Holmes LD (2012) Mass production of the beneficial nematode Heterorhabditis bacteriophora and its bacterial symbiont Photorhabdus luminescens. Ind J Microbiol 52:316–324. doi:10.1007/s12088-012-0270-2
Singh S, Eric M, Floyd I, Leonard HD (2011) Characterization of Photorhabdus luminescens growth for the rearing of the beneficial nematode Heterorhabditis bacteriophora. Ind J Microbiol 52:325–331. doi:10.1007/s12088-011-0238-7
Bai X, Adams BJ, Ciche TA, Clifton S, Gaugler R, Kim KS, Spieth J, Sternberg PW, Wilson RK, Grewal PS (2013) A lover and a fighter: the genome sequence of an entomopathogenic nematode Heterorhabditis bacteriophora. Plos One 8:e69618. doi:10.1371/journal.pone.0069618
Ciche TA, Kim KS, Kaufmann-Daszczuk B, Nguyen KC, Hall DH (2008) Cell invasion and matricide during Photorhabdus luminescens transmission by Heterorhabditis bacteriophora nematodes. Appl Environ Microbiol 74:2275–2287. doi:10.1128/AEM.02646-07
Easom CA, Joyce SA, Clarke DJ (2010) Identification of genes involved in the mutualistic colonization of the nematode Heterorhabditis bacteriophora by the bacterium Photorhabdus luminescens. BMC Microbiol 10:45. doi:10.1186/1471-2180-10-45
Lango L, Clarke DJ (2010) A metabolic switch is involved in lifestyle decisions in Photorhabdus luminescens. Mol Microbiol 77:1394–1405. doi:10.1111/j.1365-2958.2010.07300.x
Somvanshi VS, Kaufmann-Daszczuk B, Kim KS, Mallon S, Ciche TA (2010) Photorhabdus phase variants express a novel fimbrial locus, mad, essential for symbiosis. Mol Microbiol 77:1021–1038. doi:10.1111/j.1365-2958.2010.07270.x
Turlin E, Pascal G, Rousselle JC, Lenormand P, Ngo S, Danchin A, Derzelle S (2006) Proteome analysis of the phenotypic variation process in Photorhabdus luminescens. Proteomics 6:2705–2725. doi:10.1002/pmic.200500646
Yu S, Peng Y, Zheng Y, Chen W (2014) Comparative genome analysis of Lactobacillus casei: insights into genomic diversification for niche expansion. Ind J Microbiol 55:102–107. doi:10.1007/s12088-014-0496-2
Heungens K, Cowles CE, Goodrich-Blair H (2002) Identification of Xenorhabdus nematophila genes required for mutualistic colonization of Steinernema carpocapsae nematodes. Mol Microbiol 45:1337–1353. doi:10.1046/j.1365-2958.2002.03100.x
Gaudriault S, Duchaud E, Lanois A, Canoy AS, Bourot S, Derose R, Kunst F, Boemare N, Givaudan A (2006) Whole-genome comparison between Photorhabdus strains to identify genomic regions involved in the specificity of nematode interaction. J Bacteriol 188:809–814. doi:10.1128/JB.188.2.809-814.2006
Cox J, Mann M (2011) Quantitative, high-resolution proteomics for data-driven systems biology. Ann Rev Biochem 80:273–299. doi:10.1146/annurev-biochem-061308-093216
Chen S, Glazer I, Gollop N, Cash P, Argo E, Innes A, Stewart E, Davidson I, Wilson MJ (2006) Proteomic analysis of the entomopathogenic nematode Steinernema feltiae IS-6 IJs under evaporative and osmotic stresses. Mol Biochem Parasitol 145:195–204. doi:10.1016/j.molbiopara.2005.10.003
Rath A, Glibowicka M, Nadeau VG, Chen G, Deber CM (2009) Detergent binding explains anomalous SDS-PAGE migration of membrane proteins. Proc Nat Acad Sci USA 106:1760–1765. doi:10.1073/pnas.0813167106
Guan Y, Zhu Q, Huang D, Zhao S, Jan Lo L, Peng J (2015) An equation to estimate the difference between theoretically predicted and SDS PAGE-displayed molecular weights for an acidic peptide. Nat Sci Rep 5:13370. doi:10.1038/srep13370
Raymond KN, Dertz EA, Kim SS (2003) Enterobactin: an archetype for microbial iron transport. Proc Nat Acad Sci USA 100:3584–3588. doi:10.1073/pnas.0630018100
Somvanshi VS, Sloup RE, Crawford JM, Martin AR, Heidt AJ, Kim KS, Clardy J, Ciche TA (2012) A single promoter inversion switches Photorhabdus between pathogenic and mutualistic states. Science 337:88–93. doi:10.1126/science.1216641
Davies RL, Lee I (2004) Sequence diversity and molecular evolution of the heat-modifiable outer membrane protein gene (ompA) of Mannheimia (Pasteurella) haemolytica, Mannheimia glucosida, and Pasteurella trehalosi. J Bacteriol 186:5741–5752. doi:10.1128/JB.186.17.5741-5752.2004
Novak R, Cauwels A, Charpentier E, Tuomanen E (1999) Identification of a Streptococcus pneumoniae gene locus encoding proteins of an ABC phosphate transporter and a two-component regulatory system. J Bacteriol 181:1126–1133
Lutz R, Bujard H (1997) Independent and tight regulation of transcriptional units in Escherichia coli via the LacR/O, the TetR/O and AraC/I1-I2 regulatory elements. Nucleic Acids Res 25:1203–1210. doi:10.1093/nar/25.6.1203
Patridge EV, Ferry JG (2006) WrbA from Escherichia coli and Archaeoglobus fulgidus is an NAD (P) H: quinone oxidoreductase. J Bacteriol 188:3498–3506. doi:10.1128/JB.188.10.3498-3506.2006
Grunden AM, Ray RM, Rosentel JK, Healy FG, Shanmugam KT (1996) Repression of the Escherichia coli modABCD (molybdate transport) operon by ModE. J Bacteriol 178:735–744
Acknowledgments
We thank Dr. Vinay Kalia, Principal Scientist, Division of Entomology, ICAR-IARI for providing facilities for 2-D gel electrophoresis. This work was supported by Grant no. C4-3005 (Indian Council of Agricultural Research (ICAR)-National Agricultural Innovation Project); Grant no. SB/SO/AS/010/2014 (Science and Engineering Research Board, Department of Science and Technology, Government of India), and in-house funding from the Division of Nematology, ICAR-Indian Agricultural Research Institute, New Delhi.
Author information
Authors and Affiliations
Corresponding author
Ethics declarations
Conflict of interest
Authors declare no potential conflict of interest.
Human and Animal Rights
No animal and human rights were violated during this study.
Additional information
Ram Kumar and Jyoti Kushwah: equal contribution first authorship.
Sudershan Ganguly: posthumously.
Electronic supplementary material
Below is the link to the electronic supplementary material.
Rights and permissions
About this article
Cite this article
Kumar, R., Kushwah, J., Ganguly, S. et al. Proteomic Investigation of Photorhabdus Bacteria for Nematode-Host Specificity. Indian J Microbiol 56, 361–367 (2016). https://doi.org/10.1007/s12088-016-0594-4
Received:
Accepted:
Published:
Issue Date:
DOI: https://doi.org/10.1007/s12088-016-0594-4