Abstract
Moisture migration in food causes deleterious effects on food quality such as loss of crispiness in ice cream cones, drying of chocolate with liquid centers, sugar bloom and cracking of compound/chocolate coating. The present study aimed to investigate the relationship between physicochemical properties of confectionery coating fats such as fatty acids and triacylglycerol (TAG) composition, solid fat contents, rheological and crystalline properties with their moisture barrier property. Coating fats with high content of trisaturated and desaturated TAG; and high SFC at 25 °C were found to have significant (P < 0.05) positive correlation with its moisture barrier property. These fats were able to crystallize into highly crystalline materials (high SFC) with small crystal size and dense networks. Thus, these fats were able to significantly delay moisture migration. Nevertheless, some of the highly crystalline fats also had high G′ value indicating poor mechanical properties and higher tendency of such fats to crack. In order to have good moisture barrier property, coating fats need to have sufficient solids with small crystal size and dense networks; and also low G′ value. Wafer coated with good moisture barrier fat showed less cracking during storage.
Similar content being viewed by others
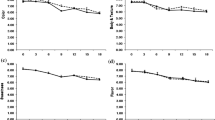
Explore related subjects
Discover the latest articles, news and stories from top researchers in related subjects.Avoid common mistakes on your manuscript.
Introduction
Foods often occur in the form of multi-domain systems such as ice cream in a cone, pastry with fruit fillings, chocolate or candy with liquid centers, chocolate- or compound-coated wafers, cheese-filled baked products, etc. [1]. Moisture migration in food happens when there is a water activity differential between the different domains within the multi-domain food system or between the food and environment. It causes deleterious effects on food quality such as loss of crispiness in ice cream cones, drying of chocolate with liquid centers, sugar bloom and cracking of compound/chocolate coating [1–4].
Moisture migration in food can be controlled through several ways. One of such method is to ensure similar water activity within the different domains in a multi-component food. This method is usually not practical as increasing water activity of a domain will lead to changes in textural properties [3]. Another method is to limit moisture migration between the food and environment through packaging; which is inefficient in preventing moisture migration between the different domains within a multi-component food [2]. A feasible solution may be applying an edible hydrophobic lipidic layer to limit and control moisture migration in foods. For example, a fat composition (≥25% SSO) which crystallizes rapidly at operating temperature (5–25 °C) has been patented for use as baked products’ coating (croissant and Danish pastry). These fat composition can prevent moisture migration and reduce cracking or sweating of the coating during storage [5].
Physicochemical properties of the lipid components in coating compositions such as fatty acid compositions and crystalline morphology; and the processing conditions used to cast the lipid-based coating have been reported to affect its water vapor permeability (WVP) and moisture barrier property [6–9]. Long chain saturated fatty acids such as stearic acids have been reported to have a higher acyl chain packing order and lower chain mobility. Meanwhile, lipids with unsaturated acyl chain and smaller head groups which have higher chain mobility demonstrated high level of packing defects [10]. High levels of packing defects can be associated with higher water vapor permeability and less efficient moisture barrier property. Duong and colleagues (2015) reported that processing conditions including cooling and shear rate also affects the fat crystal networks and subsequently moisture barrier property. Shear promoted formation of a less ordered and heterogeneous crystal size distribution comprising of small and large crystals which increased the WVP of long chain saturated fatty acids [11]. Other components in the lipid-based coating such as cocoa powder and sugar were also reported to affect the moisture barrier property of coating composition. Cocoa powder increased the water vapor permeability of lipid-based coatings; meanwhile, sugar decreased the lipid-based coating’s water vapor permeability [4].
The moisture barrier property of coating fat has been postulated to cause expansion of the coated food such as wafers and cookies; resulting in cracking of the coating composition [12]. Meanwhile, Svanberg and colleague found moisture can interact with the non-lipid components in confectionery composition such as cocoa and sugar particles, causing them to swell or dissolve resulting in mechanical and textural changes of the confectionery shell/fillings [13]. Afoakwa and colleague have comprehensively reviewed factors affecting the rheological, mechanical and textural properties of confectionery systems [14].
Chemical composition and viscoelastic properties of a good moisture barrier coating should be able to tolerate slight changes in the dimension of the coated food (e.g. swelling of wafer following moisture absorption) to avoid cracking [7].
The present study aimed to investigate the correlation between physicochemical properties of confectionery coating fats such as fatty acids and triacylglycerol composition, solid fat contents, rheological and crystalline properties with their moisture barrier property. These coating fats were mixed with cocoa powder, sugar and lecithin to form a compound coating for wafers. The relationship between the moisture barrier property of the coating fat and the tendency for compound-coated wafer to crack with changes in the relative humidity of the storage environment was also determined. To our knowledge, little work has been done to investigate the aforementioned relationship. Knowledge on the relationship between physicochemical properties of lipid components in coating compositions with a oisture barrier property will guide development of moisture barrier fat with a lower cracking tendency.
Materials and Methods
Materials
Coating fats (Fat 101, Fat 102, Fat 103, Fat 104, Fat 105, Fat 106 and Fat 107) and lecithin were obtained from by Yihai Kerry Group (Shanghai, China). Reduced fat cocoa powder (10–12% fat) was purchased from ADM (China). Sucrose and skim milk powder were purchased from a local supermarket. All chemicals and solvents used were either of analytical or high-performance liquid chromatography (HPLC) grade.
Methods
Fatty Acids Composition (FAC)
Fatty acids methyl esters (FAME) of the coating fats were prepared according to AOCS Official Method Ce 2-66 before analyzing using gas chromatography (GC) [15]. Approximately 100 mg of the fat samples were dissolved in 10 ml of hexane and mixed with 100 µl of 0.5 M methanolic potassium hydroxide. The mixture was vortexed and centrifuged to obtain a FAME-containing supernatant phase. Approximately 10 µl of the supernatant phase was diluted with 2 ml of hexane and injected into gas chromatograph (GC, 7820A, Agilent) equipped with a fused-silica capillary column (100 m × 0.25 mm × 0.2 μm) and flame ionization detector (FID). Helium was used as the carrier gas (2 ml/min). The temperatures of the injector and detector were set at 250 and 280 °C, respectively. The column temperature was programmed as follows: initial temperature of 120 °C (held for 3 min), heating at an increase of 8 °C/min to 175 °C (held for 28 min) and heating at 3 °C/min to 215 °C (held for 20 min). FAME were identified using the retention time of FAME standards and quantified based on the peak area in relative to the sum of all peak areas.
Triacylglycerols (TAG) Composition
TAG compositions of the coating fats were analyzed according to AOCS Official Method Ce 5-86 using a GC equipped with a silica capillary column (100 m × 0.25 mm × 0.2 μm) and FID [16]. Fat samples were dissolved in chloroform (10 mg/ml). Helium was used as the carrier gas (2 ml/min). The temperatures of the injector and detector were both set at 350 °C. The column temperature was initially held at 180 °C for 1 min and heated at 5 °C/min to 350 °C and kept at 350 °C for 15 min. TAG were identified according to carbon number and quantified based on the peak area in relative to the sum of all peak areas.
Slip Melting Point (SMP)
The SMP of the coating fats were determined according to AOCS Method Cc 3-25 [17]. Clean capillary tubes were first dipped in completely melted fats until the melted fats rose about 10 mm in the tubes. The capillary tubes were then held against a piece of ice until the fat solidified and kept at 5 °C for at least 16 h. Following that, the capillary tubes were attached to a thermometer using a rubber band so that the lower ends of the tubes were even with the bottom of the mercury bulb of the thermometer. Both the capillary tubes and thermometer were then suspended in a beaker containing clean distilled water. The water bath was gradually heated at a heating rate of 0.5 °C/min. The temperature at which the fat column rises in the capillary tubes were recorded as the SMP.
Solid Fat Content (SFC)
The SFC of the coating fats were measured according to AOCS Method Cd 16b-93 using pulsed nuclear magnetic resonance (Minispec 20, Bruker, Germany) [18]. NMR tubes were filled with approximately 3.5 ml of the melted coating fats and kept in a water bath at 60 °C for 30 min to eliminate all crystal memory. All tubes were then transferred to an ice-bath (0 °C) for 60 min. Finally, the NMR tubes were kept at the desired temperature (10, 20, 25, 30, 35 and 40 °C) for 30 min. After 30 min incubation time, SFC for all the samples were measured.
Crystal Microstructure
The crystal microstructures of the coating fats were observed using a polarized light microscope (Nikon E600, Japan) equipped with a CCD color video camera (Canon) at room temperature. The fat samples were completely melted by heating at 60 °C for 30 min. Approximately 10 µl of melted sample was place on top of the glass slide and covered with a coverslip. The slide was then placed on the temperature stage and subjected to crystallization conditions mimicking crystallization of compound coating in a cooling tunnel. The melted sample was first rapidly cooled to 5 °C (within 2 min). The sample was then allowed to crystallize at 5 °C for 40 min. Following that, the sample was rapidly heated to 15 °C (within 2 min) and kept at 15 °C for 20 min. The micrograph was taken at ×500 magnification.
Polymorphism Analysis
The polymorphism of the coating fats was determined using a powder X-ray diffractometer (RigakuMSC Inc., The Woodlands, TX). The coating fat samples were first completely melted by heating at 60 °C for 30 min. The melted samples were then rapidly cooled to 5 °C (within 2 min) and allowed to crystallize at 5 °C for 40 min. Following that, the sample was rapidly heated to 15 °C (within 2 min) and kept at 15 °C for 20 min. Crystallized fats were loaded onto glass holders with a sample well (0.5 mm depression). A graphite monochromator was placed between sample and detector. Angular scans was performed at 1° per min from 1° to 30° using a Cu source X-ray tube (k = 1.54 Å). MDI Jade 6.5 software was used to analyze the data obtained.
Rheological Properties
Rheological properties of the coating fats were measured using a Rheomater (Physica MCR 301, Anton Paar Co.). The experiments were carried out using a stainless steel parallel plate (20 mm diameter). The coating fats were placed on the stainless steel parallel plate (PP50) and the gap was set at 1 mm approximately for all samples. Samples were firstly heated to 60 °C to melt the sample completely and subjected to crystallization conditions mimicking crystallization of compound coating in a cooling tunnel. The melted sample was first rapidly cooled to 5 °C (within 2 min). The sample was then allowed to crystallize at 5 °C for 40 min. Following that, the sample was rapidly heated to 15 °C (within 2 min) and kept at 15 °C for 20 min. A constant frequency of 1 Hz was used and strain values were controlled from 0.001 to 10%. Following completion of the crystallization process, rheological properties of the coating fats were then measured at room temperature (25 °C). Oscillatory tests (small deformation) were performed by means of a strain sweep experiment.
Water Vapor Permeability (WVP)
The WVP of the coating fats were determined according to previously described methods [6, 19] with some modifications. The set-up used to evaluate the WVP of coating fats involves formation of a thin lipid film (usually <5 mm) as coating for a high humidity gel. This set-up is then subjected to storage in a low humidity environment. During the storage period, a set-up having a lipid with good barrier properties will demonstrate constant weight. In contrast, a set-up having a lipid with poor barrier properties will demonstrate weight loss. The high humidity gel was prepared by mixing 4.5 g of gelatin, 45 g of glycerol (85%) and 100 g of potassium sorbate solution (1 ppm). The mixture was heated to 70 °C and stirred to form a homogenous solution. The liquid melt was then poured into a transparent plastic container (36 mm × 58 mm) and allowed to solidify at room temperature. Melted coating fats were then poured on top of the high humidity gel. The temperature of the melted coating fats were controlled at approximately 40–50 °C to prevent melting of the high humidity gel. The fat-coated gels were immediately subjected to crystallization at the following conditions to mimic crystallization of compound coating in cooling tunnel (5–8 °C for 30 min; 10–15 °C for 15 min). These ensembles were stored in sealed desiccators with controlled relative humidity (RH) at room temperature (20 °C). The RH of the sealed desiccators was fixed at 3.6%. Samples were weighed at the beginning of the experiment and after different periods of time (24, 48, 72, 96 h). WVP was determined from triplicate experiments:
The WVP is calculated as
where WVTR is the water vapor transmission rate (g days−1cm−2), ΔX is the thickness of the film (mm) and ΔP is the vapor pressure difference between both sides of the film (mmhg). Therefore, the units for WVP are g mm day−1cm−2mm Hg−1.
Water vapor transmission rate can be calculated using the following equation:
where slope is the slope of the plot of weight loss vs time (g days−1) and A is the area of the film used (cm2). Fats having a good moisture barrier property will have low WVP; while fats having a poor moisture barrier property will have high WVP.
Cracking Tendency of Compound-coated Wafer
Table 1 shows the ingredients used to produce compound coating. All the ingredients were mixed and ball-milled using a Wiener ball miller for 40 min to ensure the molten compound coating had a particle size of less than 20 µ. The molten compound coating was then used to coat wafers previously equilibrated in an oven with RH of 55% at a temperature of 25 °C. The compound-coated wafers were crystallized in a temperature chamber under the following conditions (5–8 °C for 30 min; 10–15 °C for 15 min). The compound-coated wafers were finally stored in a sealed desiccator with controlled RH of 3.6% at room temperature (20 °C). The compound coated wafers were monitored and evaluated for the degree of cracking using optical microscopy. The degree of cracking is quantified as crack surface area over total surface area.
Statistical Analysis
Statistical differences were determined using one-way ANOVA (Fisher’s test) at a 95% confidence level for an individual error rate in pairwise comparison using Minitab software (Version 16, Minitab Inc., State College, PA, USA). The physicochemical parameters studied were correlated to the water vapor permeability of the fat films using Spearman’s correlation analysis using Minitab software. (Version 16, Minitab Inc., State College, PA, USA). All the crystallization experiments were conducted in triplicate.
Results and Discussion
Fatty Acid Composition
Table 2 shows the FAC of the moisture barrier fats. Fats 101, 102, 103 and 104 were lauric-containing fats with 28.2, 21.5, 14.4 and 7.5% of medium chain fatty acids, respectively. Meanwhile, fats 105, 106 and 107 were non-lauric fats with less than 1.5% of medium chain fatty acids. The non-lauric fats were high in palmitic (~38–50%) and oleic (~17–35%) acids. Non-lauric fats contained a higher ratio of long chain to medium chain fatty acids (>70) (Fig. 2b). All barrier fats contained at least 50% of saturated fatty acids (SAFA). Lauric-containing fats contained higher ratio of SAFA to unsaturated fatty acids (UFA) as compared to and non-lauric fats. The ratios of SAFA to UFA for lauric-containing fats and non-lauric fats were between 1.5 to 3.5 and 1.1 to 1.5, respectively.
Triacylglycerols (TAG) Composition
Table 3 shows the TAG composition of the moisture barrier fats. All moisture barrier fats had a complex TAG composition. Lauric-containing fats contained more medium-chain TAG. The amount of TAG with equivalent carbon number (ECN) less than 44 in fats 101, 102, 103 and 104 were 50.7, 43.1, 31.1 and 18.3%, respectively. Meanwhile, non-lauric fats contained more long chain TAG. All non-lauric fats (fats 105, 106 and 107) had less than 10% of TAG with an ECN less than 44. The major TAG in non-lauric fats were TAG with an ECN of 50 (1,3-dipalmitate 2-oleate glycerol, POP) and ECN 52 (1,2-Dioleoyl-1-Palmitoyl-Glycerol, POO) which accounted for at least 40% of the total TAG. Fats 101, 102 and 103 had high contents of trisaturated (S3) TAG (>30%). Meanwhile, fats 104, 105 106 and 107 had high contents of symmetrical TAG either in the form of disaturated (S2U) or diunsaturated (SU2) TAG (>65%).
Slip Melting Points and Solid Fat Content
Figure 1 shows the slip melting points (SMP) and solid fat contents (SFC) of the moisture barrier fats. Non-lauric fat (Fat 105) which had a high content of SAFA particularly palmitic acid (54.4%), S3 and S2U TAG (72%); had the highest SMP of 49.4 °C and lowest ratio of SFC at 25–40 °C (Fig. 1a). Meanwhile, fat 106 and 106 which had higher amount of SU2 TAG (>25%) had lower SMP and higher ratio of SFC at 25–40 °C. Despite that, the differences are not significant indicating the oral melting properties of these non-lauric fats were relatively poor.
Meanwhile, lauric-containing fats namely fats 101, 102 and 103 had SMP of less than 40 °C and high ratio of SFC at 25–40 °C (>8) (Fig. 1a). These three fats also had a steep SFC profile with less than 5% solid fats at 40 °C (Fig. 1b). Thus, these fats are expected to have good oral meltdown. Although lauric-containing fats also contained high amounts of SAFA (>65%), S3 and S2U TAG, their fatty acids were in the form of both medium and long chain fatty acids. The ratio of long chain to medium chain fatty acids for all lauric-containing fats are relatively low (<10%) (Table 2). As the melting points (MP) for medium chain SAFA are relatively lower than long chain SAFA (MP of Lauric Acid: 43.8 °C; MP of Palmitic Acid: 62.9 °C; MP of Stearic Acid: 69.3 °C) fats with a high ratio of medium to long chain fatty acids have a steeper melting profile.
Microstructural Properties
Figure 2 shows the microstructural properties of the barrier fat. Fats 101, 102, 103 and 104 which had high amounts of S3 and S2U TAG crystallized into a highly organized crystalline structure with small platelet-like crystals and a dense network. The void between such crystals was less. Meanwhile, fats 105, 106 and 107 which contained higher amounts of S2U and SU2 TAG formed larger crystals with larger void between the crystals. All the barrier fats with the exception of fat 101 crystallized into a mixture of β′ and β crystals. Fat 101 crystallized into β′ crystals.
Rheological Properties
Figure 3 shows the G′ and Tan δ values of the moisture barrier fats. Most of the fats with the exception of fat 102 had a Tan δ value of above 0.15. Low Tan δ value indicates that fat 102 had a more solid-like behavior. G′ values of the barrier fats varied significantly (P < 0.05). Fats 103, 104, 105 and 106 had low G′ values of less than 10 kPa. Fat 107 had a medium G′ values of 29 kPa. Meanwhile, both fats 101 and 102 had the highest G′ values of 41.6 and 46.8 kPa, respectively. Fat with low G′ and high Tan δ values have been reported to be more malleable as less force is required to produce permanent deformation in the structure [6]. As Tan δ values for coating fats used in the present study did not vary significantly, fats 103 (G′ = 2.4 kPa; Tan δ value = 0.209), 104 (G′ = 0.47 kPa; Tan δ value = 0.164), 105 (G′ = 0.16 kPa; Tan δ value = 0.163) and 106 (G′ = 8.05 kPa; Tan δ value = 0.197) which had low G′ values are expected to be more malleable and less likely to crack. The present study found no significant correlation between SFC/SMP with rheological properties. All of these fats demonstrated a shared characteristic of having SU2 TAG of 16–25%. It is worth noting that fat 105 which had high SMP and the lowest ratio of SFC at 25 to 40 °C demonstrated malleability probably due to the presence of both S3 and SU2 TAG.
WVP
Figure 4 shows the WVP of moisture barrier fats. Fats 103, 104, 105 and 106 had the lowest WVP indicating good moisture barrier property. Meanwhile, fats 102 and 107 showed intermediate moisture barrier ability. Fat 101 had the highest WVP indicating poor moisture barrier property. Although Fat 101 demonstrated a highly organized crystalline structure, it has a high G′ and high Tan δ values indicating it has a high tendency to crack. Thus, it is possible that Fat 101 has developed fine cracks during the crystallization process resulting in high WVP.
Relationship Between the Physicochemical Properties and WVP of the Moisture Barrier Fat
The WVP of a lipid has been reported to depend largely on its physicochemical composition including fatty acid composition, polymorphism and crystal size [6, 7, 13]. Long chain SAFA particularly palmitic and stearic acids have been reported as having a better moisture barrier in comparison to the short/medium chain and UFA due to the tighter lateral packing of the acyl chain. Tighter lateral packing of the acyl chain decreased hydrocarbon chain mobility which reduces water transport across the different domain within multi-component food system or between food and environment [6, 20]. Nevertheless, the present study found no significant correlation between the WVP and the fatty acids composition. Moisture barrier fats evaluated in the present study had a stearic and palmitic acids content of less than 10% and between 40 and 50%, respectively. The effect of palmitic and stearic acids on the WVP of different moisture barrier fats was not significant.
There was a significant (P < 0.05) correlation between WVP and TAG composition. S3 TAG were found to be positively correlated with WVP indicating high content of S3 TAG resulted in high WVP and a poor moisture barrier property. Fats with high S3 TAG (e.g. Fats 101 and 102) crystallized faster and contained higher solid fat content at room temperature (Fig. 1b). In agreement with aforementioned finding and discussion, present study found (P < 0.05) a significant positive correlation between WVP and solid fat content at 25 °C. Our findings were contrary to previously reported findings of Martini and colleagues (2006a) who reported that a highly crystalline material (high SFCmax) with a highly organized structure was able to delay moisture migration. Nevertheless, the moisture barrier property of highly crystalline fats has also been reported to decrease with increases in SFC until a threshold after which mechanical properties of the fat deteriorate [9]. Therefore, one of the possible reasons for the poor moisture barrier property in highly crystalline fats examined in the present study is the poor mechanical properties and higher cracking tendency of these highly crystalline fats. The present study found a significant (P < 0.05) positive correlation between WVP and G′ values. Highly crystalline fats with high G′ values have been reported to have poor mechanical properties [8]. Thus, barrier fats with high crystalline materials in the present study were more likely to crack due to the high G′ values resulting in a poor moisture barrier property.
Although inconclusive, crystallization behavior and polymorphism have been reported to affect the WVP of lipid barrier. β crystals have been reported to have better moisture barrier as it has dense and highly ordered crystalline structure. On the other hand, α crystals have also been reported to have a good moisture barrier property due to their flexibility which limits formation of defects during crystallization [21]. As all the moisture barrier fats examined crystallized into either β′ (d ~ 4.13 Å; d ~ 3.81 Å) or β′ and β (d ~ 4.6 Å) forms, polymorphism did not play a major role in affecting the moisture barrier property. In terms of crystal size, smaller crystals that formed a denser network with fewer voids has been reported to be able to obtain a lower WVP and prevent/delay moisture migration [7]. Nevertheless, the present study found crystals’ size and network were not the only determining factors affecting WVP. Although fats 101 and 102 formed small crystals with dense network, they showed a high WVP probably due to their poor mechanical properties.
Relationship Between the WVP and Cracking Tendency of the Moisture Barrier Fat
There was a significant (P < 0.05) correlation between the WVP and the cracking tendency of the moisture barrier fats. Fats 101, 102 and 107 which showed high WVP produced a higher amount of cracks (>8% of the total surface area) on the compound-coated wafer. Meanwhile, fats 103, 104, 105 and 106 which showed lower WVP values produced a lower amount of cracks (<8% of the total surface area) on the compound-coated wafer.
Conclusion
Suitable moisture barrier fats are able to manage and lessen moisture migration in food. The present study found a significant (P < 0.05) correlation between the physicochemical properties of barrier fats (S3 and S2U TAG; SFC at 25 °C; G′ and Tan δ values) with their moisture barrier properties. Fats with a high content of S3 and S2U were able to crystallize into highly crystalline materials (high SFC) with small crystal size and dense networks. The amount of crystalline materials must be carefully controlled so that there does not exist a threshold that results in poor mechanical properties of the fats. Highly crystallized fats with low G′ and high Tan δ values are more malleable and less likely to crack; hence demonstrating a better moisture barrier property.
References
Labuza TP, Hyman CR (1998) Moisture migration and control in multi-domain foods. Trends Food Sci Tech 9(2):47–55
Biquet B, Labuza TP (1988) Evaluation of the moisture permeability characteristics of chocolate films as an edible moisture barrier. J Food Sci 53(4):989–998
Ghosh V, Ziegler GR, Anantheswaran RC (2005) Moisture migration through chocolate-flavored confectionery coatings. J Food Eng 66(2):177–186
Ghosh V, Duda JL, Ziegler GR, Anantheswaran RC (2004) Diffusion of moisture through chocolate-flavoured confectionery coatings. Food Bioprod Process 82(1):35–43
Kumie K, Toshihiro S, Kazusue M (2007) Coating oil- and fat- composition JP2007319043 (A)
Martini S, Kim DA, Ollivon M, Marangoni AG (2006) The water vapor permeability of polycrystalline fat barrier films. J Agric Food Chem 54(5):1880–1886
Martini S, Kim DA, Ollivon M, Marangoni AG (2006) Structural factors responsible for the permeability of water vapor through fat barrier films. Food Res Int 39(5):550–558
Bourlieu C, Guillard V, Ferreira M, Powell H, Vallès-Pàmies B, Guilbert S, Gontard N (2009) Effect of cooling rate on the structural and moisture barrier properties of high and low melting point fats. J Am Oil Chem Soc 87(2):133–145
Morillon V, Debeaufort F, Blond G, Capelle M, Voilley A (2010) Factors affecting the moisture permeability of lipid-based edible films: a review. Crit Rev Food Sci 42(1):67–89
Holthuis JCM, Menon AK (2014) Lipid landscapes and pipelines in membrane homeostasis. Nature 510(7503):48–57
Duong Q, Purgianto A, Maleky F (2015) Dynamics of moisture diffusivity in solid triacylglycerol matrices. Food Res Int 75:131–139
Barron LF (1977) The expansion of wafer and its relation to the cracking of chocolate and ‘bakers’ chocolate’ coatings. J Food Tech 12:73–84
Svanberg L, AhrnÉ L, LorÉN N, Windhab E (2012) A method to assess changes in mechanical properties of chocolate confectionery systems subjected to moisture and fat migration during storage. J Texture Stud 43(2):106–114
Afoakwa EO, Paterson A, Fowler M (2007) Factors influencing rheological and textural qualities in chocolate—a review. Trends Food Sci Tech 18(6):290–298. doi:10.1016/j.tifs.2007.02.002
AOCS (1998) Official methods Ce 2-66. Official method and recommended practice of the American Oil Chemists’ Society, 5th edn. AOCS Press, Champaign
AOCS (1998) Official methods Ce 5-86. Official method and recommended practice of the American Oil Chemists’ Society, 5th edn. AOCS Press, Champaign
AOCS (1998) Official methods Cc 16b–93. Official method and recommended practice of the American Oil Chemists’ Society, 5th edn. AOCS Press, Champaign
AOCS (1998) Official methods Cd 16b-93. Official method and recommended practice of the American Oil Chemists’ Society, 5th edn. AOCS Press, Champaign
Svanberg L, Ahrné L, Lorén N, Windhab E (2013) Impact of pre-crystallization process on structure and product properties in dark chocolate. J Food Eng 114(1):90–98
Ghosh V, Ziegler GR, Anantheswaran RC (2002) Fat, moisture, and ethanol migration through chocolates and confectionary coatings. Crit Rev Food Sci 42(6):583–626
Kester JJ, Fennema O (1989) The influence of polymorphic form on oxygen and water vapor transmission through lipid films. J Am Oil Chem Soc 66(8):1147–1153
Author information
Authors and Affiliations
Corresponding author
About this article
Cite this article
Cheong, LZ., Shen, Q., Chen, F. et al. Relationship Between Physicochemical Properties and Moisture Barrier Property of Confectionery Coating Fats. J Am Oil Chem Soc 94, 425–433 (2017). https://doi.org/10.1007/s11746-017-2957-3
Received:
Revised:
Accepted:
Published:
Issue Date:
DOI: https://doi.org/10.1007/s11746-017-2957-3