Abstract
Forest productivity is closely linked to seasonal variations and vertical differentiation in leaf traits. However, leaf structural and chemical traits variation among co-existing species, and plant functional types within the canopy are poorly quantified. In this study, the seasonality of leaf chlorophyll, nitrogen (N), and phosphorus (P) were quantified vertically along the canopy of four major tree species and two types of herbs in a temperate deciduous forest. The role of shade tolerance in shaping the seasonal variation and vertical differentiation was examined. During the entire season, chlorophyll content showed a distinct asymmetric unimodal pattern for all species, with greater chlorophyll levels in autumn than in spring, and the timing of peak chlorophyll per leaf area gradually decreased as shade tolerance increased. Chlorophyll a:b ratios gradually decreased with increasing shade tolerance. Leaf N and P contents sharply declined during leaf expansion, remained steady in the mature stage and decreased again during leaf senescence. Over the seasons, the lower canopy layer had significantly higher chlorophyll per leaf mass but not chlorophyll per leaf area than the upper canopy layer regardless of degree of shade tolerance. However, N and P per leaf area of intermediate shade-tolerant and fully shade-tolerant tree species were significantly higher in the upper canopy than in the lower. Seasonal variations in N:P ratios suggest changes in N or P limitation. These findings indicate that shade tolerance is a key feature shaping inter-specific differences in leaf chlorophyll, N, and P contents as well as their seasonality in temperate deciduous forests, which have significant implications for modeling leaf photosynthesis and ecosystem production.
Similar content being viewed by others
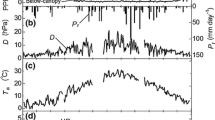
Avoid common mistakes on your manuscript.
Introduction
Photosynthetic capacity is closely related to leaf traits, such as nitrogen (N) content spatially (Ollinger et al. 2008; Osnas et al. 2013) and chlorophyll content (Chlleaf) seasonally (Noda et al. 2015; Croft et al. 2017). Under natural conditions, the most pronounced seasonal patterns of leaf N and Chlleaf occurred in temperate deciduous broadleaf forests (Croft et al. 2020). Chlorophyll content represents the physiological state of plants and is directly related to photosynthesis (Croft et al. 2017). Tree species in temperate forests have different seasonal variations in leaf nutrient and Chlleaf contents as influenced by leaf age, phenological stage, and climatic factors (Heaton et al. 2009; De Las Heras et al. 2017). In general, a dynamic change of leaf nutrient content is the result of genetic factors, phenological period and external environment (Legner et al. 2014). However, interspecific differences in the seasonal dynamics of leaf nutrient and Chlleaf levels and their intrinsic controls is poorly defined, which limits understanding of the life history responses of coexisting tree species and the accurate simulation of seasonal gross primary production (GPP).
Temperate deciduous tree species reach a peak or stable leaf area and N and phosphorous (P) levels earlier in the spring than Chlleaf, which begins to decrease earlier in the autumn than leaf area and N (Noda et al. 2015; Yang et al. 2016; Wang et al. 2020; Zhuang et al. 2021; Chen et al. 2022b). For example, in the Harvard Forest in the United States, leaf area index (LAI) reached a maximum within 10 days after bud break, but Chlleaf (expressed with chlorophyll normalized difference index) of the dominant species, Quercus rubra L. took longer to maximize, and began to decline 40 days earlier than LAI in the autumn (Keenan et al. 2014). The asynchrony between Chlleaf and Nleaf can be identified by variations in the ratio of chlorophyll to nitrogen (Chl: N). The peak dates of Chl: N ratio differed by about 10 days in the spring and 20 days in the autumn among the four major tree species in the Borden mixed temperate Forest (Croft et al. 2017). However, the differences in asynchrony between Chlleaf and Nleaf among tree species remain unclear, which hinders our understanding of the seasonality of leaf N allocation and thus forest GPP.
Nutrient allocation within the canopy is considered optimal for maximizing growth, which depends mainly on soil nutrient availability and canopy structure (Nilsen and Abrahamsen 2003; Niinemets 2007; Mandre 2009; Kobayashi et al. 2010; Yoshimura 2010). Canopy structure determines the light environment within the canopy, which influences the transport of canopy nutrients (Mandre 2009; Ots et al. 2009). The canopy is considered spatially heterogeneous in leaf traits (Granata et al. 2020). Generally, lower canopy leaves are larger and thinner, and have lower leaf mass per area (LMA) which enhances the net carbon gain of the whole canopy (Legner et al. 2014). Nitrogen is redistributed to different photosynthetic components and affects the photosynthetic potential of the leaves (Niinemets et al. 2015). N and P levels were higher in the upper part of the canopy in some studies (Finer 1994; Sabate et al. 1995; Liu et al. 2012), but were comparable between vertical levels of the canopy in other studies (Hollinger 1996; Yoshimura 2010). In addition, Ots et al. (2009) found that N content was higher in the lower part of the canopy, but P levels did not differ essentially between canopy layers, indicating an element-dependence of vertical difference. However, these discrepancies between studies are unclear. Shade tolerance may play an important role in shaping many leaf structural and chemical traits (Valladares and Niinemets 2008), and vertical variations of nutrient and chlorophyll contents, with shade tolerant species showing greater vertical variation in leaf traits (Wang et al. 2013; Legner et al. 2014). In addition, larger canopies of overstory species may negatively impact shade tolerant species (Coble and Cavaleri 2015) depending on canopy development stage across the seasons. However, few studies have quantified the differences in chlorophyll and nutrient contents between different coexisting shade-tolerant species for the entire growing season.
East Asia has one of the three major temperate forest zones in the world and play an important role in regional carbon cycling. In this study, five species and one species group with different shade tolerance from a deciduous broadleaf forest in northeast China were selected to investigate seasonal dynamics and interspecific differences in chlorophyll and nutrient contents. The specific objectives were to: (1) reveal seasonal patterns of leaf chlorophyll and nutrient contents in trees and herbs; (2) quantify differences in chlorophyll and nutrient contents between the upper and lower canopies of different species over the growing season; and (3) explore the relationship of chlorophyll and nutrient contents with degree of shade tolerance. It was hypothesized that: (1) leaf chlorophyll and nutrient contents of temperate trees and herbs would show asymmetric seasonal patterns; (2) chlorophyll and nutrient contents would differ significantly between canopy layers; and (3) degree of shade tolerance would have significant effects on chlorophyll and nutrient levels.
Material and methods
Site description
The study was carried out at the Maoershan Forest Ecosystem Research Station of the Northeast Forestry University, Northeast China (45°24′N, 127°40′E). The climate is continental monsoonal, with warm and humid summers and cold, dry winters. Records for 2008 to 2019 indicated that mean temperatures were 2.1 ± 0.8 °C and mean annual precipitation 726 ± 261 mm (Sun et al. 2021). The main vegetation is a typical natural secondary forest of the mountainous areas of eastern northeast China.
Experimental design
The sampling sites were located around the flux towers at an average elevation of 400 m (Liu et al. 2021). The main species or species group were (shade-intolerant white birch (Betula platyphylla Suk.), intermediate shade tolerant Manchurian ash (Fraxinus mandshurica Rupr.), shade-tolerant Japanese elm (Ulmus davidiana var. japonica (Rehder) Nakai), subcanopy tree species clove (Syringa reticulata var. mandshurica (Maxim.) H.Hara) (Sun et al. 2023), evergreen horsetail (Equisetum hyemale L.) and a group of broad-leaved herbs. Due to space limitation, only the differences among species and between canopy layers were reported in this paper, and the up-scaled results on chlorophyll, N and P levels at the ecosystem scale to interpret the seasonality of GPP will be reported in a following paper.
Based on phenological characteristics (Liu et al. 2015), the growing season in 2018 was divided into three periods, i.e., leaf expansion (day135–day153), maturity (day154–day245), and senescence (day246–day278). Samples were taken every ten days during leaf expansion and maturity periods, and every five days during senescence. Three individuals were selected for each tree species in typical habitats. Sampling was carried out on rain-free days between 8:00 and 16:00 but avoiding noon time. For each sampling, one branch with a diameter of ≥ 3 cm was cut from the upper canopy and in the lower half for birch, ash, and elm. Leaves were randomly sampled from each branch and randomly from the canopy for clove due to the short canopy length. Leaves of horsetail are greatly reduced and non-photosynthetic, and photosynthesis is carried out by the stems. Horsetail and broad-leaved herbs were cut from three 1-m2 plots. The samples were sealed and stored in a cooler and taken to the laboratory for the determination of leaf/stem structural and chemical traits. A total of 1548 samples were obtained, including 334 from birch, 283 from ash, 370 from elm, 166 from clove, 223 from horsetail, and 172 from broad-leaved herbs. Leaf trait data were represented by the average of three samples, and for each tree species in each sampling time by the average of three individuals.
Sample treatment
Leaf chlorophyll content
Chlleaf was determined by a 1:1 mixture of 80% acetone and 95% anhydrous ethanol as the extracting solution. One hundred mg of fresh leaves was cut into fine fragments and moved into a test tube containing 10 mL of extracting solution at room temperature (10–30 °C) in the dark until the leaf fragments became transparent, usually after a few hours or days. The extracting solution was then transferred to a 25-mL flask for volume determination. The absorbance of chlorophyll extracts at 663 nm and 645 nm was measured on a spectrophotometer (UV–VIS, Purkinje General Instrument Co., Beijing, China) with three replicates of each sample. The contents of chlorophyll a (Chl a), chlorophyll b (Chl b) and total chlorophyll were calculated with Arnon’s formula (Lichtenthaler 1987):
where A663 and A645 are the absorbances of the chlorophyll solution at wavelengths of 663 nm and 645 nm, respectively; Chl a (mg g−1) and Chl b (mg g−1) are the contents of chlorophyll a and chlorophyll b, respectively, and Chlmass (mg g−1) is the total Chlleaf. The Chlleaf per unit dry leaf mass was obtained by correcting for leaf water content. Chlorophyll per leaf area (Chlarea, μg cm−2) was calculated as the product of Chlmass and leaf mass per area. The ratio of Chl a to Chl b (Chl a:b) was calculated to compare the strategies of shade tolerance and response to drought (Kitajima and Hogan 2003).
Leaf nutrient content
Leaf samples were treated at 105 °C for 30 min to eliminate enzymatic activity, dried at 70 °C for 48 h, and ground to pass a 0.2-mm sieve. The solution to be measured was prepared by the H2SO4-H2O2 decoction method, and total nitrogen (Nmass, mg g−1) and total phosphorus (Pmass, mg g−1) were determined by a continuous flow analyzer (SEAL Analytical GmbH, Germany) (Wang et al. 2022).
Nitrogen per leaf area (Narea, g m−2) and phosphorus per leaf area (Parea, g m−2) were calculated from corresponding nutrient mass content by multiplying the LMA. Thirty leaves from each sample were randomly selected to measure the LMA, the ratio of leaf dry mass (g) to leaf area (m2). Leaf area was measured based on leaf pixels versus background pixels in the scanned images and scanning resolution (300 PDI). The ratio of Chlmass and Nmass (Chl:N ratio) represented N allocation to chlorophyll (Croft et al. 2017).
Data analysis
A repeated measures ANOVA was used to compare the effects of species, sampling date, and the interaction between the two on Chlleaf, and nutrient content, because the Mauchly sphericity test was significant at P < 0.05. The correlations of leaf Chlleaf and nutrient contents were investigated by Pearson's correlation analysis. All statistical analyses were done with SPSS 21, and all plots were drawn with SigmaPlot 12.5.
Results
Seasonality of chlorophyll content
Species, sampling time, and their interaction differentially affected Chlmass and Chlarea of trees and herbs (Tables 1 and 2). The Chlmass of trees was higher than that of horsetails but lower than broad-leaved herbs, while the opposite was true for Chlarea. Chlleaf differed significantly among the four tree species, with ash being highest. However, these species showed similar unimodal seasonal patterns of Chlleaf, lowest during leaf expansion, followed by a gradual increase, reaching a maximum in summer, and rapidly decreased for most tree species in senescence. For the same species, Chlmass generally peaked earlier than Chlarea (Fig. 1). Drought around day150 resulted in a slight decrease in Chlmass. Both Chlmass and Chlarea of the four tree species were seasonally asymmetric, with greater levels in autumn than in spring (Chlmass: (71.0 ± 19.3) % vs. (37.0 ± 11.4) %, Chlarea: (80.0 ± 13.3) % vs. (53.0 ± 2.6) %). Interestingly, the peak Chlarea for the four species was gradually delayed by increasing shade tolerance, with birch, ash, elm and clove on day200, day210, day225, and day250, respectively (Fig. 1). Although canopy had no significant effect on Chlarea for most species, Chlmass was significantly higher in the lower canopies of birch, ash and elm (P < 0.05).
Seasonality of chlorophyll content for different tree species. ASU and ASL are the relative amplitudes of chlorophyll in the upper and lower canopy in the spring, and AAU and AAL the relative amplitudes of chlorophyll in the upper and lower canopy in the autumn. The error bar is standard deviation. BP: Betula platyphylla, FM: Fraxinus mandshurica, UJ: Ulmus davidiana var. japonica, SM: Syringa reticulata var. mandshurica, EH: Equisetum hyemale, BH: Broad-leaved herbs. The canopy was separated into upper- and lower-canopy layers for BP, FM and UJ. Shade-intolerant species: BP, intermediate species: FM, shade-tolerant species: UJ, SM, EH, BH. The same below
The Chlmass of horsetail was significantly lower than that of broad-leaved herbs and opposite for Chlarea (Fig. 2). Both Chlmass and Chlarea of broad-leaved herbs had similar unimodal seasonal patterns as trees. In contrast, horsetail levels rebounded slightly during senescence and maximized around day150, while the maximum of broad-leaved herbs occurred around day190.
Species, sampling time, and their interaction significantly (P < 0.05) affected Chl a:b of trees (Table 1), while sampling time significantly (P < 0.05) affected Chl a:b of herbs (Table 2). As expected, the Chl a:b of birch, ash, elm, clove, and herbs gradually decreased with increasing shade tolerance. The seasonality of Chl a:b was similar among tree species: it was higher in leaf expansion, then declined rapidly and remained stable and declined again during senescence. The drought around day150 resulted in higher Chl a:b for all tree species (Fig. 1). In contrast, Chl a:b in herbs was low during leaf expansion, then suddenly increased and then decreased around the drought event. After entering maturity, it first decreased rapidly and then remained stable (Fig. 2). The Chl a:b of tree species was > 3:1 in leaf expansion, then decreased and remained at 2.1:1–2.4:1 in maturity. There were insignificant differences in Chl a:b between canopy layers. The Chl a:b of herbs was about 2.2:1 during leaf expansion, > 3:1 in maturity, and then decreased and stabilized at about 2:1.
Seasonality of N and P contents
Species had significant effects on N and P contents of leaves and horsetail stems (P < 0.05, Tables 1 and 2). Sampling date and the interaction of sampling date and species were highly significant in affecting the Narea, Pmass and Parea of trees (P < 0.05, Table 1), and sampling date had significant effects on Nmass of trees (P < 0.05, Table 1). Sampling date and its interaction with species were highly significant in affecting the Narea of herbs (P < 0.05, Table 2).
N and P levels of tree species exhibited similar seasonal dynamics. They decreased rapidly during leaf expansion, remained largely stable into maturity, and decreased rapidly again at senescence (Figs. 3 and 4). Throughout the growing season, Nmass and Narea of ash was significantly higher than that of the other three species. Ash had significantly higher Pmass than clove. In addition, the differences in P content among tree species also varied by growing season. During leaf expansion, elm had the highest P levels and clove the lowest. At maturity, P contents of the four tree species were similar, although birch had higher P at senescence (Fig. 3). Comparing values between the two canopy layers, Narea and Parea were significantly higher in the upper canopy for ash and elm but not for birch over the seasons (P < 0.05, Fig. 3).
Horsetail had significantly lower Nmass and Pmass than broad-leaved herbs, but Narea and Parea were opposite. The dynamics of N and P contents of herbs differed from that of trees. Nmass of horsetail fluctuated less throughout the growing season and was higher in during leaf expansion, then decreased during the drought, increased gradually at maturity, and decreased slightly during senescence (Fig. 4a). Narea of horsetail was lower earlier, increased rapidly and decreased during maturity, and first increased and then decreased during senescence (Fig. 4c). Both Pmass and Parea of horsetail were lower during leaf expansion, increased rapidly, then decreased rapidly, increased again at maturity and decreased slightly during senescence (Figs. 4e, g). N and P contents of broad-leaved herbs showed a seasonal pattern of first decreasing, then increasing, and then decreasing again (Figs. 4b, d, f, h).
Sampling date, species, and their interaction significantly affected Chl:N ratios of trees (P < 0.05, Table 1), and time and species significantly the Chl:N ratios of herbs (P < 0.05, Table 2). The highest seasonal maximum of the Chl: N ratio was for broad-leaved herbs, followed by elm; the lowest occurred in ash. Chl:N ratio seasonality showed a similar unimodal pattern for all species. It increased rapidly during leaf expansion, reached a maximum value in summer and decreased rapidly during senescence (Fig. 5). The peak leaf Chl:N ratio of the four tree species, in order from early to late, were clove, birch, ash, and elm. Interestingly, the Chl:N ratio of ash and clove plateaued and then declined, while birch and elm peaked and then declined rapidly (Fig. 5). The Chl:N ratio in the lower ash canopy was slightly higher than that in the upper canopy, and decreased earlier (Fig. 5b). In addition, the Chl:N ratio of horsetail did not significant decrease during the senescence period (Fig. 5e).
Seasonality of N:P
Species and the interaction with sampling date significantly affected N:P ratios of trees (P < 0.05, Table 1). Ash had the highest N:P ratio, that of clove was higher than that for birch and elm. All species showed an increase during leaf expansion, but the changes were very different in maturity and senescence. The ratios of birch increased in the upper canopy, while it decreased in the lower canopy at maturity and decreased rapidly in both layers at senescence (Fig. 6a). The N:P ratio of ash and elm changed less at maturity and senescence (Fig. 6b, c). The ratios for clove increased throughout the growing season (Fig. 6d). There was a clear peak in the ratios of horsetail around day150 in response to the drought, and was relatively stable at maturity and at senescence for both horsetail (Fig. 6e) and broad-leaved herbs (Fig. 6f). Ratios did not differ significantly between the upper and lower canopy layers (Fig. 6).
Discussion
Interspecific differences in seasonal dynamics of chlorophyll and nutrients
Temperate trees and broad-leaved herbs showed similar unimodal patterns for Chlleaf (Fig. 1), consistent with the literature (Wang et al. 2013; Noda et al. 2015; Yang et al. 2016). However, our results indicate that Chlleaf peaks of herbs were earlier than that of tree species, and that of shade-intolerant species were earlier than shade-tolerant species. The Chlleaf of herbs started to decline earlier than that of tree species; the decline of Chlarea of trees gradually delayed as shade tolerance increased. In addition, shade tolerance appeared to affect the seasonal asymmetry of Chlleaf, with the shade-tolerant elm having a significantly lower Chlleaf amplitude in spring than in autumn, while the intermediate tolerant ash showed little difference between spring and autumn. Most shade-tolerant species initiate growth earlier in the season than shade-intolerant ones in order to optimize light availability, increasing photosynthetic efficiency and resulting in overall increases in GPP (Kikuzawa 2003; Valladares and Niinemets 2008). We summarized the influence of shade tolerance on leaf traits of different species and canopy layers in Table 3.
Leaf N and P levels of all temperate species in this study showed similar seasonal patterns (Fig. 3) as those of other studies (Yang et al. 2016, 2017; Chen et al. 2022a, 2022b; Seidel et al. 2022). Leaf development in the expansion stage requires large amounts of protein and nucleic acid, and thus the content of N and P is higher (Chapin and Kedrowski 1983). Subsequently, the nutrient absorption rate is lower than the growth rate of new leaves and shoots, and the dilution effect caused by the rapid growth of leaf structural components results in a decrease in nutrients (Chapin and Kedrowski 1983; Palma et al. 2000; Yang et al. 2017). After full leaf expansion, leaf biomass is stable and the nutrients in the soil are in the best effective state, which is conducive to their uptake and N and P levels remain relatively constant. At the end of the growing season, nutrient resorption during leaf senescence reduces leaf N and P contents to a minimum (Wang et al. 2022).
In addition, according to the theory of optimal N allocation, higher Narea is more beneficial for shade-intolerant species (Hallik et al. 2009b; Hikosaka 2016). Except for ash, Narea was lower for shade-tolerant clove and elm during leaf expansion. As shade tolerance increased, Narea and Parea declined more rapidly during leaf expansion. The shade-intolerant birch was the earliest to begin senescence. In contrast, ash had a significantly shorter apoptosis than the other species and ended apoptosis earlier (Wang et al. 2022). N and P content per unit leaf area of the understory evergreen horsetail was lower in the early spring than in other seasons, consistent with the understory evergreen shrub spotted laurel, Aucuba japonica Thunb. (Muller et al. 2011). Clearly, tolerance to low light conditions, rather than height acquisition and N conversion, is the growth strategy established by understory plants (Hallik et al. 2009a).
Increasing the proportion of N allocated to chlorophyll is another strategy of adaption to low light conditions (Kitaoka and Koike 2004; Muller et al. 2005; Katahata et al. 2007; Zhuang et al. 2021). Under the canopy, the carbon gain of plants can be improved by capturing more light (Niinemets 2010). Therefore, efficient light harvesting under natural conditions is essential for plants growing in dense stand competition (Valladares and Niinemets 2008). Remobilizing N from leaves under the canopy and allocating them to leaves exposed to higher light can enhance the carbon gain of leaves (Niinemets 2023). The allocation of N among different photosynthetic components varied with canopy layer (Zhuang et al. 2021). The leaves of shade-tolerant species are generally more efficient at resource use than those of shade-intolerant species (Legner et al. 2014). Under low light conditions, to improve and optimize light harvesting, shade-tolerant species allocate more N to light-harvesting chlorophyll complex proteins, while less shade-tolerant species allocate more N to a key photosynthetic enzyme, Rubisco, and electron transport components (Niinemets and Tenhunen 1997; Evans and Poorter 2001; Bachofen et al. 2020). In shade intolerant species, electron transport capacity is optimized in terms of light availability, while for shade-tolerant species, carboxylation capacity is optimized (Legner et al. 2014). In this study, Chl:N ratios of broad-leaved herbs were significantly higher than those of other species, but those of tree species did not increase with shade tolerance (Fig. 5). Croft et al. (2017) showed that, compared with other tree species on the same site, shade-intolerant aspens (Populus grandidentata Michx. and P. tremuloides Michx.) had lower Chl:N ratios and consequently their Chlleaf and nutrient contents were higher. This may because aspen allocated more N into RuBisCo rather than the light harvesting pigment protein complex, thus increasing photosynthetic capacity. Compared with other tree species, ash has lower Chl:N ratios and higher chlorophyll and nutrient contents, showing similar N partitioning as aspen (Croft et al. 2017), which may lead to a higher photosynthetic capacity than other tree species at this site. As expected, Chl a:b gradually decreased with increasing shade tolerance and was greater in tree species than in herbs, reflecting a light-gradient adaptation among co-existing species (Li et al. 2018).
Canopy leaf N:P ratios may indicate a relative nutrient limitation status (Koerselman and Meuleman 1996). In terms of annual mean leaf N:P ratios, ash was P limited and the other species N limited (Fig. 6), which is consistent with the general overall pattern of N limitations in temperate regions (Du et al. 2020). However, there were distinct changes in nutrient limitations for different phenological phases. The increasing leaf N:P ratios of clove suggest a progressive P deficiency throughout the growing season, corresponding to a higher P resorption efficiency (41.2%) than N resorption efficiency (11.1%) at this site (Wang et al. 2022). The generally lower values of leaf N:P ratios and their rapid decrease during senescence of birch leaves corresponded to higher N resorption efficiency (41.6%) than P resorption (27.2%) (Wang et al. 2022). In addition, the trend in N:P ratios of senescing leaves (Fig. 6) was consistent with that of leaf litter (Wang et al. 2022) for the four study species, indicating that they equally indicate the relative limitation of leaf N and P. However, there was no shade tolerance effect on the seasonality of N:P ratios.
Differences in chlorophyll and nutrient content between canopy layers
The theory of optimal N allocation indicates that Chlmass of the lower leaves is higher than the upper leaves, and that Chlarea is slightly lower than the upper leaves. Nitrogen is a major element of photosynthetic enzymes. Upper leaves are allocated more N because more light is available (Bachofen et al. 2020; Zhuang et al. 2021). Further, upper canopy leaves under intense light conditions can avoid damage with lower Chlleaf and higher Chl a:b ratios (Niinemets 2007; Lichtenthaler and Babani 2022). However, lower leaves contain a greater proportion of chlorophyll and carotenoid light-harvesting pigments and reduce the Chl a:b ratios to intercept more light (Kitajima and Hogan 2003; Kenzo et al. 2006; Niinemets 2007; Lichtenthaler and Babani 2022).
Shade tolerance also affects leaf N and P contents between canopy layers. N and P levels of the shade intolerant birch were not significantly affected by position in the canopy, while the Narea and Parea were higher in the upper canopy than in the lower canopy for the shade tolerant elm and the intermediate tolerant ash, in agreement with previous studies (Sabate et al. 1995; Liu et al. 2012; Wang et al. 2021). On the one hand, this may be related to interspecific differences and the micro-environment (Wang et al. 2021). High soil N can reduce the dependence of birch on the light environment (Yoshimura 2010). On the other hand, the coexistence of broad-leaved tree species also affects nutrient acquisition and utilization strategies (Legner et al. 2014). Nitrogen in shade tolerant species should be more sensitive to reduced irradiance than shade intolerant ones since increasing the mass of light harvesting compounds per leaf and the specific absorptivity of shaded leaves are strategies to reduce photosynthesis and light limitation (Niinemets and Tenhunen 1997). Legner et al. (2014) also showed that the Nmass in the lower canopy was higher than in the upper part. Therefore, the vertical Narea gradient is considered an adaptive strategy for efficient N use (Hirose 2005; Niinemets et al. 2015), and was related to shade tolerance of species.
Implications for estimating chlorophyll and nutrient content at an ecosystem scale
The vertical and interspecific differentiation in chlorophyll and nutrient contents found in this study has considerable implications for up-scaling from leaf to canopy level. The protocol for standardized measurement of plant functional traits to sample sun leaves for the fairest comparison across individuals or species (Perez-Harguindeguy et al. 2013), cannot satisfy the scaling up from individual measurements to ecosystem traits (He et al. 2019). Chlarea, Narea and Parea tend to be slightly higher in sun leaves than in shaded ones (Figs. 1, 3) (Zhang et al. 2007), thus scaling up based on upper leaf chlorophyll and nutrients and LAI would overestimate canopy chlorophyll and nutrients (Simic et al. 2011; Croft et al. 2015; Li et al. 2021), particularly for shade tolerant species. This overestimation may be further amplified in autumn, considering that the Chlarea of lower canopy leaves begins to decrease earlier in autumn for some species such as intermediate shade tolerant ash and shade tolerant elm in this study, and another shade tolerant species, Cryptomeria japonica (Thunb. ex L.f.) D. Don in a Japanese temperate forest (Kobayashi et al. 2010). This potential error may explain the mismatch between canopy chlorophyll and GPP before and after day250 in Croft et al. (2015), in which only sunny leaves were sampled.
Co-existing tree species are not likely synchronized in spring leaf area expansion (Liu et al. 2015) nor in abscission in the autumn (Wang et al. 2022). Therefore, an accurate estimation of leaf area or biomass seasonality of different tree species is an issue that must be considered for up-scaling. Unfortunately, previous studies adopted the proportion of tree species composition as a fixed sharing factor of canopy LAI for the corresponding species across the growing season (e.g., Simic et al. 2011; Croft et al. 2015; Li et al. 2021), i.e., the issue that leaf phenology is out-of-sync among co-existing species was ignored.
The seasonality of chlorophyll and nutrient contents of the evergreen horsetail differed significantly from deciduous trees. The seasonal dynamics of broad-leaved herbs were closely similar to those of deciduous trees, although their leaf expansion was earlier and senescence later (Liu et al. 2019). Unfortunately, most ecosystem-scale estimates of chlorophyll and nutrient contents in forest ecosystems have not considered herbaceous plants, which would underestimate absolute values in the spring and autumn.
Conclusion
In this temperate deciduous broadleaved forest, the leaf chlorophyll content of trees and broad-leaved herbs had similar asymmetric unimodal seasonal dynamics. The peak of chlorophyll content and the decline of chlorophyll/area were gradually delayed as shade tolerance increased. The chlorophyll/leaf mass of the lower canopy was significantly higher than that of the upper canopy, independent of degree of shade tolerance. Leaf nutrient contents of trees and broad-leaved herbs also showed similar seasonal patterns. Nitrogen/leaf area and phosphorous/leaf area decreased more rapidly in shade tolerant species during leaf development. Intermediate and shade tolerant species had significantly higher nitrogen and phosphorous per leaf area in the upper canopy. These results highlight that shade tolerance plays a key role on seasonality and vertical differentiation of leaf nutrients and chlorophyll, which will help accurately estimate chlorophyll and nutrient contents at an ecosystem scale.
Abbreviations
- N:
-
Nitrogen
- P:
-
Phosphorus
- Chl:
-
Chlorophyll
- Chl a:
-
Chlorophyll a content
- Chl b:
-
Chlorophyll b content
- N leaf :
-
Leaf nitrogen
- N mass :
-
Nitrogen/leaf mass
- N area :
-
Nitrogen/leaf area
- P leaf :
-
Leaf phosphorus
- P mass :
-
Phosphorus/leaf mass
- P area :
-
Phosphorus/leaf area
- Chlleaf :
-
Leaf chlorophyll
- Chlmass :
-
Chlorophyll/leaf mass
- Chlarea :
-
Chlorophyll/leaf area
References
Bachofen C, D’Odorico P, Buchmann N (2020) Light and VPD gradients drive foliar nitrogen partitioning and photosynthesis in the canopy of European beech and silver fir. Oecologia 192(2):323–339. https://doi.org/10.1007/s00442-019-04583-x
Chapin FS, Kedrowski RA (1983) Seasonal changes in nitrogen and phosphorus fractions and autumn retranslocation in evergreen and deciduous taiga trees. Ecology 64(2):376–391. https://doi.org/10.2307/1937083
Chen JM, Wang R, Liu YH, He LM, Croft H, Luo XZ, Wang H, Smith NG, Keenan TF, Prentice IC, Zhang YG, Ju WM, Dong N (2022a) Global datasets of leaf photosynthetic capacity for ecological and earth system research. Earth Syst Sci Data 14(9):4077–4093. https://doi.org/10.5194/essd-14-4077-2022
Chen LT, Zhang Y, Nunes MH, Stoddart J, Khoury S, Chan AHY, Coomes DA (2022b) Predicting leaf traits of temperate broadleaf deciduous trees from hyperspectral reflectance: Can a general model be applied across a growing season? Remote Sens Environ 269:112767. https://doi.org/10.1016/j.rse.2021.112767
Coble AP, Cavaleri MA (2015) Light acclimation optimizes leaf functional traits despite height-related constraints in a canopy shading experiment. Oecologia 177(4):1131–1143. https://doi.org/10.1007/s00442-015-3219-4
Croft H, Chen JM, Froelich NJ, Chen B, Staebler RM (2015) Seasonal controls of canopy chlorophyll content on forest carbon uptake: implications for GPP modeling. J Geophys Res: Biogeo 120(8):1576–1586. https://doi.org/10.1002/2015JG002980
Croft H, Chen JM, Luo X, Bartlett P, Chen B, Staebler RM (2017) Leaf chlorophyll content as a proxy for leaf photosynthetic capacity. Global Change Biol 23(9):3513–3524. https://doi.org/10.1111/gcb.13599
Croft H, Chen JM, Wang R, Mo G, Luo S, Luo X, He L, Gonsamo A, Arabian J, Zhang Y, Simic-Milas A, Noland T, He Y, Homolová L, Malenovský Z, Yi Q, Beringer J, Amiri R, Hutley L, Arellano P, Stahl C, Bonal D (2020) The global distribution of leaf chlorophyll content. Remote Sens Environ 236:111479. https://doi.org/10.1016/j.rse.2019.111479
De Las Heras J, Hernández-Tecles EJ, Moya D (2017) Seasonal nutrient retranslocation in reforested Pinus halepensis Mill. stands in Southeast Spain. New for 48:397–413. https://doi.org/10.1007/s11056-016-9564-2
Du E, Terrer C, Pellegrini AF, Ahlström A, van Lissa CJ, Zhao X, Xia N, Wu X, Jackson RB (2020) Global patterns of terrestrial nitrogen and phosphorus limitation. Nat Geosci 13(3):221–226. https://doi.org/10.1038/s41561-019-0530-4
Evans JR, Poorter H (2001) Photosynthetic acclimation of plants to growth irradiance: the relative importance of specific leaf area and nitrogen partitioning in maximizing carbon gain. Plant Cell Environ 24(8):755–761. https://doi.org/10.1046/j.1365-3040.2001.00724.x
Finer L (1994) Variation in needle nutrient concentrations in the crown of Scots pine on peatland. Silva Fenn 28(1):41–51. https://doi.org/10.14214/sf.a9161
Granata MU, Bracco F, Nola P, Catoni R (2020) Photosynthetic characteristic and leaf traits variations along a natural light gradient in Acer campestre and Crataegus monogyna. Flora 268:151626. https://doi.org/10.1016/j.flora.2020.151626
Hallik L, Kull O, Niinemets Ü, Aan A (2009a) Contrasting correlation networks between leaf structure, nitrogen and chlorophyll in herbaceous and woody canopies. Basic Appl Ecol 10(4):309–318. https://doi.org/10.1016/j.baae.2008.08.001
Hallik L, Niinemets U, Wright IJ (2009b) Are species shade and drought tolerance reflected in leaf-level structural and functional differentiation in Northern Hemisphere temperate woody flora? New Phytol 184(1):257–274. https://doi.org/10.1111/j.1469-8137.2009.02918.x
He NP, Liu CC, Piao SL, Sack L, Xu L, Luo YQ, He JS, Han XG, Zhou GS, Zhou XH, Lin Y, Yu Q, Liu SR, Sun W, Niu SL, Li SG, Zhang JH, Yu GR (2019) Ecosystem traits linking functional traits to macroecology. Trends Ecol Evol 34(3):200–210. https://doi.org/10.1016/j.tree.2018.11.004
Heaton EA, Dohleman FG, Long SP (2009) Seasonal nitrogen dynamics of Miscanthus × giganteus and Panicum virgatum. GCB Bioenergy 1(4):297–307. https://doi.org/10.1111/j.1757-1707.2009.01022.x
Hikosaka K (2016) Optimality of nitrogen distribution among leaves in plant canopies. J Plant Res 129(3):299–311. https://doi.org/10.1007/s10265-016-0824-1
Hirose T (2005) Development of the Monsi-Saeki theory on canopy structure and function. Ann Bot-London 95(3):483–494. https://doi.org/10.1093/aob/mci047
Hollinger DY (1996) Optimality and nitrogen allocation in a tree canopy. Tree Physiol 16(7):627–634. https://doi.org/10.1093/treephys/16.7.627
Katahata SI, Naramoto M, Kakubari Y, Mukai Y (2007) Photosynthetic capacity and nitrogen partitioning in foliage of the evergreen shrub Daphniphyllum humile along a natural light gradient. Tree Physiol 27(2):199–208. https://doi.org/10.1093/treephys/27.2.199
Keenan TF, Darby B, Felts E, Sonnentag O, Friedl MA, Hufkens K, O’Keefe J, Klosterman S, Munger JW, Toomey M, Richardson AD (2014) Tracking forest phenology and seasonal physiology using digital repeat photography: a critical assessment. Ecol Appl 24(6):1478–1489. https://doi.org/10.1890/13-0652.1
Kenzo T, Ichie T, Watanabe Y, Yoneda R, Ninomiya I, Koike T (2006) Changes in photosynthesis and leaf characteristics with tree height in five dipterocarp species in a tropical rain forest. Tree Physiol 26(7):865–873. https://doi.org/10.1093/treephys/26.7.865
Kikuzawa K (2003) Phenological and morphological adaptations to the light environment in two woody and two herbaceous plant species. Funct Ecol 17(1):29–38. https://doi.org/10.1046/j.1365-2435.2003.00707.x
Kitajima K, Hogan KP (2003) Increases of chlorophyll a/b ratios during acclimation of tropical woody seedlings to nitrogen limitation and high light. Plant Cell Environ 26(6):857–865. https://doi.org/10.1046/j.1365-3040.2003.01017.x
Kitaoka S, Koike T (2004) Invasion of broad-leaf tree species into a larch plantation: seasonal light environment, photosynthesis and nitrogen allocation. Physiol Plantarum 121(4):604–611. https://doi.org/10.1111/j.1399-3054.2004.00359.x
Kobayashi H, Inoue S, Gyokusen K (2010) Spatial and temporal variations in the photosynthesis-nitrogen relationship in a Japanese cedar (Cryptomeria japonica D. Don) canopy. Photosynthetica 48(2):249–256. https://doi.org/10.1007/s11099-010-0031-6
Koerselman W, Meuleman AFM (1996) The vegetation N: P ratio: a new tool to detect the nature of nutrient limitation. J Appl Ecol 33(6):1441–1450. https://doi.org/10.2307/2404783
Legner N, Fleck S, Leuschner C (2014) Within-canopy variation in photosynthetic capacity, SLA and foliar N in temperate broad-leaved trees with contrasting shade tolerance. Trees 28(1):263–280. https://doi.org/10.1007/s00468-013-0947-0
Li Y, He NP, Hou JH, Xu L, Liu CC, Zhang JH, Wang QF, Zhang X, Wu XQ (2018) Factors influencing leaf chlorophyll content in natural forests at the biome scale. Fron Ecol Evol 6:10. https://doi.org/10.3389/fevo.2018.00064
Li YJ, Ma QM, Chen JM, Croft H, Luo XZ, Zheng T, Rogers C, Liu J (2021) Fine-scale leaf chlorophyll distribution across a deciduous forest through two-step model inversion from sentinel-2 data. Remote Sens Environ 264:112618. https://doi.org/10.1016/j.rse.2021.112618
Lichtenthaler HK (1987) Chlorophylls and carotenoids: pigments of photosynthetic biomenbranes. Method Enzymol 148:350–382. https://doi.org/10.1016/0076-6879(87)48036-1
Lichtenthaler HK, Babani F (2022) Contents of photosynthetic pigments and ratios of chlorophyll a/b and chlorophylls to carotenoids (a+b)/(x+c) in C4 plants as compared to C3 plants. Photosynthetica 60:3–9. https://doi.org/10.32615/ps.2021.041
Liu JX, Zhang DQ, Zhou GY, Duan HL (2012) Changes in leaf nutrient traits and photosynthesis of four tree species: effects of elevated [CO2], N fertilization and canopy positions. J Plant Ecol 5(4):376–390. https://doi.org/10.1093/jpe/rts006
Liu ZL, Wang CK, Chen JM, Wang XC, Jin GZ (2015) Empirical models for tracing seasonal changes in leaf area index in deciduous broadleaf forests by digital hemispherical photography. Forest Ecol Manag 351:67–77. https://doi.org/10.1016/j.foreco.2015.05.005
Liu F, Wang XC, Wang CK (2019) Measuring vegetation phenology with near-surface remote sensing in a temperate deciduous forest: effects of sensor type and deployment. Remote Sensing 11(9):1063. https://doi.org/10.3390/rs11091063
Liu F, Wang XC, Wang CK, Zhang QZ (2021) Environmental and biotic controls on the interannual variations in CO2 fluxes of a continental monsoon temperate forest. Agric for Meteorol 296:108232. https://doi.org/10.1016/j.agrformet.2020.108232
Mandre M (2009) Vertical gradients of mineral elements in Pinus sylvestris crown in alkalised soil. Environ Monit Assess 159(1–4):111–124. https://doi.org/10.1007/s10661-008-0616-8
Muller O, Hikosaka K, Hirose T (2005) Seasonal changes in light and temperature affect the balance between light harvesting and light utilisation components of photosynthesis in an evergreen understory shrub. Oecologia 143(4):501–508. https://doi.org/10.1007/s00442-005-0024-5
Muller O, Hirose T, Werger MJ, Hikosaka K (2011) Optimal use of leaf nitrogen explains seasonal changes in leaf nitrogen content of an understorey evergreen shrub. Ann Bot-London 108(3):529–536. https://doi.org/10.1093/aob/mcr167
Niinemets Ü (2007) Photosynthesis and resource distribution through plant canopies. Plant Cell Environ 30(9):1052–1071. https://doi.org/10.1111/j.1365-3040.2007.01683.x
Niinemets Ü (2010) A review of light interception in plant stands from leaf to canopy in different plant functional types and in species with varying shade tolerance. Ecol Res 25(4):693–714. https://doi.org/10.1007/s11284-010-0712-4
Niinemets Ü (2023) Variation in leaf photosynthetic capacity within plant canopies: optimization, structural, and physiological constraints and inefficiencies. Photosynth Res 158:131–149. https://doi.org/10.1007/s11120-023-01043-9
Niinemets Ü, Tenhunen JD (1997) A model separating leaf structural and physiological effects on carbon gain along light gradients for the shade-tolerant species Acer saccharum. Plant Cell Environ 20(7):845–866. https://doi.org/10.1046/j.1365-3040.1997.d01-133.x
Niinemets Ü, Keenan TF, Hallik L (2015) A worldwide analysis of within-canopy variations in leaf structural, chemical and physiological traits across plant functional types. New Phytol 205(3):973–993. https://doi.org/10.1111/nph.13096
Nilsen P, Abrahamsen G (2003) Scots pine and Norway spruce stands responses to annual N, P and Mg fertilization. Forest Ecol Manag 174(1):221–232. https://doi.org/10.1016/S0378-1127(02)00024-5
Noda HM, Muraoka H, Nasahara KN, Saigusa N, Murayama S, Koizumi H (2015) Phenology of leaf morphological, photosynthetic, and nitrogen use characteristics of canopy trees in a cool-temperate deciduous broadleaf forest at Takayama, central Japan. Ecol Res 30(2):247–266. https://doi.org/10.1007/s11284-014-1222-6
Ollinger SV, Richardson AD, Martin ME, Hollinger DY, Frolking SE, Reich PB, Plourde LC, Katul GG, Munger JW, Oren R, Smith ML, Paw UKT, Bolstad PV, Cook BD, Day MC, Martin TA, Monson RK, Schmid HP (2008) Canopy nitrogen, carbon assimilation, and albedo in temperate and boreal forests: functional relations and potential climate feedbacks. P Natl Acad Sci USA 105(49):19336–19341. https://doi.org/10.1073/pnas.0810021105
Osnas JLD, Lichstein JW, Reich PB, Pacala SW (2013) Global leaf trait relationships: mass, area, and the leaf economics spectrum. Science 340(6133):741–744. https://doi.org/10.1126/science.1231574
Ots K, Mandre M, Pärn H, Kask R, Pikk J (2009) Changes in the allocation of nutrients and biomass in scots pine (Pinus sylvestris L.) canopy in an area of cement industry in Northeast Estonia. Balt for 15(2):237–247
Palma RM, Defrieri RL, Tortarolo MF, Prause J, Gallardo JF (2000) Seasonal changes of bioelements in the litter and their potential return to green leaves in four species of the argentine subtropical forest. Ann Bot-London 85(2):181–186. https://doi.org/10.1006/anbo.1999.1005
Perez-Harguindeguy N, Diaz S, Garnier E, Lavorel S, Poorter H, Jaureguiberry P, Bret-Harte MS, Cornwell WK, Craine JM, Gurvich DE, Urcelay C, Veneklaas EJ, Reich PB, Poorter L, Wright IJ, Ray P, Enrico L, Pausas JG, de Vos AC, Buchmann N, Funes G, Quetier F, Hodgson JG, Thompson K, Morgan HD, ter Steege H, van der Heijden MGA, Sack L, Blonder B, Poschlod P, Vaieretti MV, Conti G, Staver AC, Aquino S, Cornelissen JHC (2013) New handbook for standardised measurement of plant functional traits worldwide. Aust J Bot 61(3):167–234. https://doi.org/10.1071/BT12225
Sabate S, Sala A, Gracia CA (1995) Nutrient content in Quercus ilex canopies: seasonal and spatial variation within a catchment. Plant Soil 169(1):297–304. https://doi.org/10.1007/BF00029341
Seidel F, Lopez CML, Bonifacio E, Kurokawa H, Yamanaka T, Celi L (2022) Seasonal phosphorus and nitrogen cycling in four Japanese cool-temperate forest species. Plant Soil 472(1–2):391–406. https://doi.org/10.1007/s11104-021-05251-x
Simic A, Chen JM, Noland TL (2011) Retrieval of forest chlorophyll content using canopy structure parameters derived from multi-angle data: the measurement concept of combining nadir hyperspectral and off-nadir multispectral data. Int J Remote Sens 32(20):5621–5644. https://doi.org/10.1080/01431161.2010.507257
Sun XF, Liu F, Zhang QZ, Li YC, Zhang LF, Wang J, Zhang HY, Wang CK, Wang XC (2021) Biotic and climatic controls on the interannual variation in canopy litterfall of a deciduous broad-leaved forest. Agric for Meteorol 307:108483. https://doi.org/10.1016/j.agrformet.2021.108483
Sun X, Wang XC, Wang CK, Zhang QZ, Guo QX (2023) Filling the “vertical gap” between canopy tree species and understory shrub species: biomass allometric equations for subcanopy tree species. J Forestry Res 34(4):903–913. https://doi.org/10.1007/s11676-022-01568-0
Valladares F, Niinemets Ü (2008) Shade tolerance, a key plant feature of complex nature and consequences. Annu Rev Ecol Evol S 39(1):237–257. https://doi.org/10.1146/annurev.ecolsys.39.110707.173506
Wang L, Ibrom A, Korhonen JFJ, Arnoud Frumau KF, Wu J, Pihlatie M, Schjoerring JK (2013) Interactions between leaf nitrogen status and longevity in relation to N cycling in three contrasting European forest canopies. Biogeosciences 10(2):999–1011. https://doi.org/10.5194/bg-10-999-2013
Wang SQ, Li Y, Ju WM, Chen B, Chen JH, Croft H, Mickler RA, Yang FT (2020) Estimation of leaf photosynthetic capacity from leaf chlorophyll content and leaf age in a subtropical evergreen coniferous plantation. J Geophys Res Biogeo 125(2):14. https://doi.org/10.1029/2019jg005020
Wang CS, Guo JJ, Zhao ZG, Wang H, Zeng J (2021) Spatial patterns and seasonal dynamics of foliar nutrients in 5-year-old Betula alnoides plantations. Forest Ecol Manag 480:118683. https://doi.org/10.1016/j.foreco.2020.118683
Wang XC, Song HM, Liu F, Quan XK, Wang CK (2022) Timing of leaf fall and changes in litter nutrient concentration compromise estimates of nutrient fluxes and nutrient resorption efficiency. Forest Ecol Manag 513:120188. https://doi.org/10.1016/j.foreco.2022.120188
Yang X, Tang JW, Mustard JF, Wu J, Zhao KG, Serbin S, Lee JE (2016) Seasonal variability of multiple leaf traits captured by leaf spectroscopy at two temperate deciduous forests. Remote Sens Environ 179:1–12. https://doi.org/10.1016/j.rse.2016.03.026
Yang HL, Yang X, Heskel M, Sun SC, Tang JW (2017) Seasonal variations of leaf and canopy properties tracked by ground-based NDVI imagery in a temperate forest. Sci Rep 7(1):1267. https://doi.org/10.1038/s41598-017-01260-y
Yoshimura K (2010) Irradiance heterogeneity within crown affects photosynthetic capacity and nitrogen distribution of leaves in Cedrela sinensis. Plant Cell Environ 33(5):750–758. https://doi.org/10.1111/j.1365-3040.2009.02100.x
Zhang YQ, Chen JM, Thomas SC (2007) Retrieving seasonal variation in chlorophyll content of overstory and understory sugar maple leaves from leaf-level hyperspectral data. Can J Remote Sens 33(5):406–415. https://doi.org/10.5589/m07-037
Zhuang J, Zhou L, Wang YL, Chi YG (2021) Nitrogen allocation regulates the relationship between maximum carboxylation rate and chlorophyll content along the vertical gradient of subtropical forest canopy. Agric for Meteorol 307:108512. https://doi.org/10.1016/j.agrformet.2021.108512
Acknowledgements
We thank Guangdong Cao and Daosheng Liang for their help in collecting samples, and the Maoershan Forest Ecosystem Research Station for logistic support.
Author information
Authors and Affiliations
Corresponding author
Additional information
Publisher's Note
Springer Nature remains neutral with regard to jurisdictional claims in published maps and institutional affiliations.
Project funding: This work was supported by the National Natural Science Foundation of China (32171765).
The online version is available at http://www.springerlink.com.
Corresponding editor: Yanbo Hu.
Supplementary Information
Below is the link to the electronic supplementary material.
Rights and permissions
Springer Nature or its licensor (e.g. a society or other partner) holds exclusive rights to this article under a publishing agreement with the author(s) or other rightsholder(s); author self-archiving of the accepted manuscript version of this article is solely governed by the terms of such publishing agreement and applicable law.
About this article
Cite this article
Zeng, J., Liu, F., Zhu, Y. et al. Degree of shade tolerance shapes seasonality of chlorophyll, nitrogen and phosphorus levels of trees and herbs in a temperate deciduous forest. J. For. Res. 35, 72 (2024). https://doi.org/10.1007/s11676-024-01703-z
Received:
Accepted:
Published:
DOI: https://doi.org/10.1007/s11676-024-01703-z