Abstract
The treatment of cooking oil wastewater is an urgent issue need to be solved. We aimed to screen for efficient oil-degrading bacteria and develop a new microbial agent for degrading waste cooking oil in oily wastewater. Three extremely effective oil-degrading bacteria, known as YZQ-1, YZQ-3, and YZQ-4, were found by the enrichment and acclimation of samples from various sources and separation using oil degradation plates. The 16S rRNA sequencing analysis and phylogenetic tree construction showed that the three strains were Bacillus tropicus, Pseudomonas multiresinivorans, and Raoultella terrigena. Under optimal degradation conditions, the maximal degradation rates were 67.30 ± 3.69%, 89.65 ± 1.08%, and 79.60 ± 5.30%, respectively, for YZQ-1, YZQ-3, and YZQ-4. Lipase activity was highest for YZQ-3, reaching 94.82 ± 12.89 U/L. The best bacterial alliance was obtained by adding equal numbers of microbial cells from the three strains. Moreover, when this bacterial alliance was applied to oily wastewater, the degradation rate of waste cooking oil was 61.13 ± 7.30% (3.67% ± 2.13% in the control group), and COD removal was 62.4% ± 5.65% (55.60% ± 0.71% in the control group) in 72 h. Microbial community analysis results showed YZQ-1 and YZQ-3 were adaptable to wastewater and could coexist with local bacteria, whereas YZQ-4 could not survive in wastewater. Therefore, the combination of YZQ-1 and YZQ-3 can efficiently degrade oil and shows great potential for oily wastewater treatment.
Graphical Abstract

Similar content being viewed by others
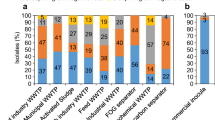
Explore related subjects
Discover the latest articles, news and stories from top researchers in related subjects.Avoid common mistakes on your manuscript.
Introduction
China discharges over 60 million tons of kitchen waste annually (Gao et al. 2019). With the rapid development of the catering and food industries, the discharge of oily wastewater has increased remarkably (Tong et al. 2021). Oily kitchen wastewater mainly originates from housing societies, restaurants, workplaces, canteens, and markets (Awasthi et al. 2018; Le et al. 2022). Furthermore, a large amount of untreated sewage is discharged directly into the urban water pipe network, resulting in severe environmental pollution. Establishing sewage discharge standards is a relatively underdeveloped field (Cheng et al. 2017). The treatment of grease in domestic sewage has always been the focus of research (Ktata et al. 2020). Lipid accumulation in wastewater leads to drainage system blockage, unpleasant odour generation, and the death of aquatic organisms (Mongkolthanaruk and Dharmsthiti 2002). Oily wastewater from restaurants and kitchens contains several macromolecular substances, such as animal and vegetable oils and proteins, and the content of organic substances is exceptionally high (Sarac and Ugur 2016). In addition, it has high salt and chemical oxygen demand (COD) concentrations (Chandrasekhar et al. 2022; Xu et al. 2022). This has led to a significant increase in the difficulty of treating sewage.
Waste cooking oil (WCO) is composed of fatty acids and glycerine. They are challenging to break down and get rid of since they are nearly insoluble in water (Ahmad et al. 2020). Treatment methods for oily wastewater include physical, chemical, and biological processes. However, physical and chemical methods are expensive and can only remove suspended, emulsified, and dispersed oil (Nimkande and Bafana 2022). This intricate process produces secondary pollutants quickly (Montagnolli et al. 2009). In recent years, biological treatment and bioremediation technologies have become research hotspots in wastewater treatment because of their high efficiency, economy, ecological friendliness, and other characteristics (Wang et al. 2022; Zahri et al. 2021b). Various biological methods have been used to jointly treat sewage and biodegradable solid waste (Rajpal et al. 2014; Xing et al. 2011). Biological processes can remove the dissolved oil and most other organic matter so that the oily wastewater can reach the discharge standards (Sutar et al. 2023; Zou 2015). Recently, researchers have developed a novel way of dealing with this problem, i.e., the use of oil-degrading bacteria. There have been increasing studies on screening and isolating microbial strains with high oil degradation efficiency (Affandi et al. 2014; Nzila et al. 2017b; Sharma et al. 2019). Most of these studies characterised the ability of oil-degrading bacteria to process simulated oily wastewater in the laboratory. More research is needed on their degradation potential. Therefore, screening for oil-degrading bacteria that affect natural oily wastewater is essential. Serratia marcescens showed the maximum oil degradation ability (91%) after incubation for 12 days (Affandi et al. 2014). Three mixed cultures of Pseudomonas aeruginosa, Acinetobacter calcoaceticus, and Bacillus sp. reduced the oil content in wastewater from 210000 mg/L to 20 mg/L within 12 days (Mongkolthanaruk and Dharmsthiti 2002). Although oil was degraded, the treatment duration was excessive, and the degradation efficiency was slow. Therefore, it is necessary to screen strains with high oil degradation ability in oily wastewater.
To address the above problems, this study screened three strains of highly efficient oil-degrading bacteria from oily wastewater. Their identification and degradation characteristics were researched, respectively. They were optimized and compounded to form a bacterial alliance and applied to the treatment of the oily wastewater. In addition, the changes in the microbial community during the wastewater treatment process were analyzed to verify the role of bacterial alliance.
Materials and methods
Chemicals and media
Luria–Bertani (LB) medium (yeast extract 5.0 g/L, tryptone 10.0 g/L, NaCl 10.0 g/L), enrichment (EM) medium (yeast extract 5.0 g/L, tryptone 10.0 g/L, NaCl 10.0 g/L, soybean oil 20.0 g/L), select (SM) medium (KH2PO4 0.3 g/L, MgSO4·7H2O 0.1 g/L, K2HPO4 1.5 g/L, (NH4)2SO4 1.0 g/L, NaCl 5.0 g/L, soybean oil 3.0 g/L, neutral red indicator, agar 18 g/L), degradation (DM) medium (Ke et al. 2021) (KH2PO4 0.3 g/L, MgSO4·7H2O 0.1 g/L, K2HPO4 1.5 g/L, (NH4)2SO4 1.0 g/L, NaCl 5.0 g/L, soybean oil 3.0 g/L, yeast extract 5.0 g/L), lipase fermentation (LFM) medium (yeast extract 20.0 g/L, CaSO4·2H2O 0.1 g/L, MgSO4·7H2O 0.1 g/L, glucose 20.0 g/L, KH2PO4 0.5 g/L).
Isolation and identification of oil-degrading strains
The source of strain separation used in this experiment was oily wastewater from the Jixian Canteen in the East Lake Campus of Zhejiang A and F University. The collected samples were placed in a centrifuge tube sterilised using ultraviolet light and stored in a—20 ℃ refrigerator until use. The sample (5 mL) was diluted to 100 mL with EM and cultured in a constant temperature oscillating incubator at 30 ℃, 150 rpm, every 7 d as a cycle. From this culture medium, 1 mL was added to fresh EM. When the oil was degraded, 2 g of soybean oil was added. Four cycles were performed for the above process. The acclimated bacterial solution was removed, diluted in a gradient, coated on SM, and cultivated in a constant temperature incubator at 30 ℃ for 24 h. During this period, red colonies growing in the SM were picked up, and streaked on to isolate a single strain. An inoculation ring was used to pick up the isolated single colony. This colony was cultured in 100 mL of DM and incubated for 72 h in a constant-temperature oscillating incubator at 30 °C and 160 rpm. The control group was set up at the same time. The oil content in the culture medium was determined using ultraviolet spectrophotometry, and strains with high oil degradation rates were isolated (Ren et al. 2021).
The selected strains were divided into LB medium and cultured at 30 °C in a constant temperature incubator for 24 h. The bacteria fluid was centrifuged at 12000 rpm for 5 min, and 2.5% glutaraldehyde solution (2 mL) was added to the centrifuge tube (Yang et al. 2022). The samples were sent to the Electron Microscope Center of Zhejiang University, and the morphology of the bacteria was observed using transmission electron microscopy. The screened strains were sent to Zhejiang Youkang Biotechnology Co., Ltd. for 16S rRNA gene sequencing analysis. The 16S rRNA gene of the bacterium was sequenced with 27F (5′-GTTTGATCCTGGCTCAG-3′) and 1492R (5′- TACGGCTACCTTGTTACGACTT-3′), and a segment of the target strain sequence was obtained (Yang et al. 2023). The obtained sequences were searched and compared using BLAST in the NCBI database, and 16S rRNA sequences with high similarity to a particular sequence were screened (Datta et al. 2018). A phylogenetic tree was constructed using MEGA7.0 to find the strain with the closest homology.
Determination of oil degradation rate
The degradation rate of soybean oil was determined by UV spectrophotometry (Mannu et al. 2022; Matwijczuk et al. 2018). 1 g of soybean oil was dissolved in a 100 mL volumetric flask, diluted to the standard line with petroleum ether. The mother liquor containing a 10 mg/mL soybean oil standard solution was prepared. Then we prepared soybean oil solutions with concentrations of (0.25, 0.50, 0.75, 1.00, 1.50, 2.00) mg/mL, respectively. A standard curve was plotted by measuring the absorbance value of the soybean oil standard solution at the above mass concentrations with petroleum ether as a blank solution at 225 nm using ultraviolet spectrophotometry. The oil in the fermentation broth was extracted with petroleum ether, shaken, and left to stand for 30 min. The upper liquid layer was diluted appropriately to measure light absorption. The concentration of the remaining oil was calculated according to the standard curve, and the oil degradation rate was calculated using the following formula:
\(\mathrm{Oil\;degradation\;rate}=\frac{\mathrm{initial\;oil\;content\;}\left(\mathrm{g}\right)-\mathrm{\;residual\;oil\;content\;}\left(\mathrm{g}\right)}{\mathrm{initial\;oil\;content\;}\left(\mathrm{g}\right)}\times 100\)%
Degradation characteristics of isolated strains
To plot the growth curve of the strain, we inoculated a 5% (V/V) seed solution in 100 mL DM and cultivated it in a constant temperature oscillating incubator at 30 ℃ and 160 rpm. The samples were collected every 4 h. After proper dilution, the OD600 value was measured using an ultraviolet spectrophotometer, and the growth curve of the strain was drawn according to the calculated absorbance value (Li et al. 2014). About the initial pH, seed solution at 5% (V/V) was inoculated in 100 mL DM at pH of 5.0, 6.0, 7.0, 8.0, and 9.0, respectively. The strain was incubated in a constant-temperature oscillation incubator at 30 °C and 160 rpm for 72 h to explore the degradation rate of soybean oil under different pH conditions. About the culture temperature, it was set to 25 °C, 30 °C, 35 °C, 40 °C, and 45 °C, and incubated for 72 h in a constant-temperature oscillating incubator at 160 rpm. The degradation rate of soybean oil was determined at various temperatures. About the salt resistance, the initial mass concentration of NaCl was set to 0 g/L, 5.0 g/L, 10.0 g/L, 15.0 g/L, 20.0 g/L and incubated for 72 h in a constant-temperature oscillating incubator at 30 ℃ and 160 rpm. The degradation rate of soybean oil by the strain at different NaCl concentrations was determined.
Determination of lipase activity
The lipase activity of selected strains was determined using the p-nitrophenol (p-NP) method (Yoo et al. 2011). The strains were activated, inoculated into 100 mL LFM, and shaken at 160 rpm and the optimum strain temperature for 72 h. After which, 2.1 mL Tris HCl (pH = 8.0) solution, 200 μL p-nitrophenol palmitate (p-NPP) (7.5 mM, acetonitrile as solvent), and 100 μL of appropriately diluted fermentation supernatant were added into a 10 mL EP tube. After shaking the mixing system at 37 °C for 10 min, the eppendorf tube was placed in an ice bath, and 100 μL 0.1 M zinc sulfate solution was added to terminate the reaction. A 0.45 μM water membrane was used to filter the reaction solution, and the absorbance at 405 nm was measured. The lipase activity was evaluated based on the standard absorbance curve of p-nitrophenol.
Emulsifying capacity of fermentation broth
The fermentation broth was incubated for 24 h and centrifuged for 5 min at 13000 rpm (4 °C). Oil displacement test was performed. 20 mL of distilled water was added to the clean culture dish, and 100 μL of soybean oil was added to form a uniform oil film on its surface. Afterwards, 10 μL of the cell-free supernatant fermentation broth was added. A halo was formed around the supernatant droplets to indicate oil displacement activity, and the diameter of the oil drain ring was measured and recorded (Sharma and Pandey 2020). To explore whether a biosurfactant was produced in E24 without the cell supernatant, 3 mL each of cell-free supernatant fermentation broth and n-hexane were added into the colorimetric tube. Then, the tube was whirled violently for 2 min and left at 4 °C for 24 h, E24 was the percentage of the emulsion layer height to the total liquid height (Sharma et al. 2019, 2022).
Optimization combination test of strains
The three selected oil-degrading bacteria were grouped and combined, and the OD600 of each strain culture solution was adjusted to 1.0 using sterile water. The inoculation amount of each strain was mixed to obtain 2 mL of total microbial flora solution. The mixed solution was then inoculated into 100 mL DM with soybean oil, at a concentration of 20 g/L, to verify the oil degradation ability of each group at 30 °C and 160 rpm.
Application of oil-degrading bacteria combination in oily wastewater
The combination of bacteria with the highest degradation rate was connected to actual oily wastewater (sourced from the Jixian Canteen of Zhejiang Agriculture and Forestry University) to determine its oil degradation ability. The test group SY was set up by injecting bacteria solution (the proportion of three oil-degrading bacteria is 1:1:1, and the inoculation amount is 15%) into the sterilised triangular bottle containing 100 mL of actual domestic sewage (the oil content was 20 g/L), placing it in a constant temperature oscillating incubator for 72 h, the temperature of the incubator was set to 30 ℃, and the rotating speed was 160 rpm. The degradation of waste cooking oil (WCO) was determined, and samples were collected every 24 h to detect the changes in other wastewater indicators during the degradation process. The determination method of WCO degradation rate is consistent with the soybean oil in 2.3. CK was used as the control group without the bacterial solution. Other indicators included cell growth (OD600), pH, COD, total phosphorus (TP), and N-NO3−.
Statistical analysis
Most experiments were conducted in triplicate, and their respective mean values ± standard deviations are reported. The application test of oily wastewater was repeated twice. The data were analysed using Microsoft Excel and SPSS 19.0. The software Origin 2018C 64-bit was used to create all the figures in this paper.
Results and discussion
Screening and identification of oil-degrading strains
There were eight strains whose colonies turned red after 48 h of culture on the solid plate of the SM, which indicated that these eight strains could degrade oil and were numbered YZQ-1–8 in turn. They were inoculated into DM, and the inoculation amount was 5% (V/V). The mixture was incubated at 30 °C and 160 rpm for 72 h, and the oil degradation was determined. Three strains with strong degradation abilities were isolated, namely YZQ-1, YZQ-3, and YZQ-4 (Fig. 1A). The unit lipase activity was determined using the P-NP method. The P-NP standard curve is shown in Figure S3. As shown in Fig. 1B, three strains showed vigorous lipase activity, of which YZQ-3 had the highest activity, reaching 94.82 ± 12.89 U/L, and YZQ-1 and YZQ-4 had enzyme activities of 22.93 ± 0.4 U/L and 28.52 ± 5.38 U/L, respectively. Based on 16S rRNA sequence analysis and comparison, MEGA7.0 software was used to construct a phylogenetic tree. The results showed that the similarity between strain YZQ-1 and Bacillus tropicus strain AOA-CPS1 was more than 99% (Fig. 2A), the similarity between strain YZQ-3 and Pseudomonas multiresinivorans strain was more than 98% (Fig. 2B), and the similarity between strain YZQ-4 and Raoultella terrigena strain JH01 was more than 99% (Fig. 2C). YZQ-1 was round and white on LB solid medium. The morphological characteristics observed under the transmission electron microscope are shown in Fig. 3A. YZQ-3 was round and translucent on solid LB medium. The morphological characteristics observed using the transmission electron microscope are shown in Fig. 3B. YZQ-4 was round and fine on the LB solid medium. The morphological attributes observed using transmission electron microscope are shown in Fig. 3C. Biosurfactants can effectively reduce the surface tension of the oil–water mixture, thus obtaining a better oil solubility (Sharma et al. 2019). Oil expansion and emulsification were used to explore the degree of emulsification of the fermentation broth of the screened strains (Mishra et al. 2023). 1 μL of fermentation broth was introduced into a glass plate containing 100 μL of liquid paraffin oil. Its emulsification activity was determined by observing the diameter of the oil drain ring produced. Table 1 shows that the oil drainage circle created by the fermentation broth of the three strains was more remarkable than that of the CK group (4.25 ± 1.06 mm), indicating that the three strains produced a small number of biosurfactants to promote the degradation of oil. The results of the emulsification activity test confirmed this result.
Degradation characteristics of oil-degrading strains
Create the soybean oil standard curve with the experimental strategy described in 2.3 (Fig. S1). After fitting, the following calculation formula is obtained: y = 0.6414x + 0.1968 (R2 = 0.9986), “y” is absorbance, “x” is the concentration of soybean oil, and “R2” is the correlation coefficient. Extract the sample (fermentation broth) to be tested with petroleum ether, measure its absorbance value at a wavelength of 225 nm, and calculate the oil concentration using the formula.
Figure 4A shows the growth curves of the three oil-degrading strains. YZQ-4 reached its highest value in the logarithmic phase at 24 h and then began to enter the stationary phase. YZQ-1 and YZQ-3 reached their highest values in the logarithmic phase at 36 h and began to enter the stationary phase. The oil degradation process of the three strains of bacteria did not end or slow down when the number of microorganisms reached the peak of the logarithmic growth period (about 24 h). The oil degradation rate remained high at 0–72 h, but it was almost unchanged at 72–96 h. Therefore, 72 h was selected as the optimal time. pH is a crucial factor that affects the survival of microorganisms. Environmental pH affects the membrane permeability and the enzyme activity by affecting surface charge of the cell membrane and absorption process of nutrients by microorganisms, which inhibits the growth of microorganisms and eventually kills them (Zahri et al. 2021a). The enzyme activity of microorganisms was the highest, and the growth rate was the fastest under optimum pH conditions. Figure 4B shows the changes in the oil degradation rate of the three strains under different pH conditions. The optimum pH for YZQ-1, YZQ-3, and YZQ-4 was 7.0, and the degradation rates of soybean oil were 46.43 ± 5.48%, 89.65 ± 1.08%, and 59.83 ± 6.09%, respectively. These strains degraded oil at pH 5.0–9.0. It can be concluded that the strains have a wide range of pH activity and that they have a specific resistance to changes in the external environment. When the pH was lower than 7.0, the growth of the three bacterial strains was greatly affected, resulting in a decline in their ability to degrade the oil. Previous studies have also shown that most commercial oil-degrading bacteria cannot grow effectively under low-pH conditions (Matsuoka et al. 2009). YZQ-1, YZQ-3, and YZQ-4 had degradation effects on soybean oil in the range of 25–45 ℃, as shown in Fig. 4D. At 35 ℃, YZQ-1 had the highest degradation rate of soybean oil in 72 h, reaching 67.30 ± 3.69%. YZQ-3 had the highest oil degradation rate at 40 ℃, reaching 82.60 ± 11.30%. YZQ-4 had the highest oil degradation rate at 30 ℃, reaching 59.83 ± 6.09%. When the temperature is lower than the optimum temperature, the oil degradation rate shows a downward trend, indicating that the low-temperature environment inhibits the ability of the strain to proliferate and secrete lipase or that the specific activity of lipase in the low-temperature environment is reduced, which ultimately affects the oil degradation effect (Chandra et al. 2020). When the temperature was higher than the optimum temperature, the oil degradation rate by the strain also decreased gradually because the temperature promoted biochemical reactions, accelerated the proliferation of the strain, rapidly consumed nutrients, and accumulated excessive metabolites, resulting in the weakening of its metabolic capacity (Szymczak et al. 2021). These strains still degrade oil at different temperatures. The osmotic pressure of microbial cells can be maintained by simple metal ions and compounds such as Na+ and K+. High NaCl concentrations inhibit the enzyme activity of microorganisms, resulting in lower respiratory rates, growth and reproduction rates, and nutrient absorption capacities (Cao and Zhao 2009). In addition, a high-salt environment places cells in a hypertonic state, and cells are prone to dehydration and even death (Pendashteh et al. 2010; Shi et al. 2012). However, when the NaCl level is too low and the cell is in a hypotonic environment, it leads to cell enlargement, which also impairs the ability of microorganisms to absorb nutrients. The effects of different NaCl concentrations on oil degradation by the three strains are shown in Fig. 4C. The optimal NaCl concentrations for YZQ-1, YZQ-3, and YZQ-4 are 10 g/L, 5 g/L, and 15 g/L, respectively. And the corresponding degradation rates were 53.94 ± 2.61%, 89.63 ± 1.62%, and 79.60 ± 5.30%. With the increasing NaCl concentration, the water in the cells constantly permeated into the extracellular space, which affected the growth of the strain and resulted in a gradual reduction in the oil degradation rate. Without NaCl, strains YZQ-1 and YZQ-4 grew well, whereas YZQ-3 barely decomposed oil. Three strains demonstrate significant salt resistance along with rapid oil decomposition rates at high NaCl concentrations.
Optimization combination of bacteria and degradation of actual oily wastewater
Based on the formation of antibacterial circles between strains, antagonistic experiments were conducted on YZQ-1, YZQ-3, and YZQ-4. The results are shown in Table S1. There is no transparent ring between them (Fig. S4), indicating that there is no antagonism between the three strains. The three strains were divided into combinations to test their synergistic ability to degrade soybean oil. Sterile water was used to adjust the OD600 value of each culture solution to 1.0. The inoculation rate of different culture solutions was 1:1. As shown in the Fig. 5A, the soybean oil degradation rate using a combination of the three strains was the highest, reaching 97.11 ± 2.22%, which was significantly better than that of single strains and other combinations. Therefore, a microbial community composed of YZQ-1, YZQ-3, and YZQ-4 is best for WCO degradation.
(A) Oil degradation rate of different strains combination. (B) Change of wastewater pH in degradation test of oily wastewater. (C) Change of OD600 of wastewater in degradation test of oily wastewater. (D) Change of COD of wastewater in degradation test of oily wastewater. (Notes: "SY" indicates the experimental group containing three combined bacterial, "CK" indicates the control group without adding isolated bacterium.)
According to the standard curve fitting of WCO (Fig. S2), the following calculation formula is obtained: y = 0.588x + 0.2838 (R2 = 0.995), “y” is absorbance, “x” is the concentration of WCO, and “R2” is the correlation coefficient. After extracting the sample (fermentation broth) with petroleum ether, measure its absorbance value at a wavelength of 225 nm, and then calculate the concentration of WCO using the formula. In the biodegradation test of oily wastewater, the changes in pH, OD600, COD concentration, N-NO3− concentration, and TP primarily reflected the growth and working state of the strain (Zhou et al. 2021). Because of the low concentration of N-NH4+ and N-NO2−, a study is yet to be conducted in this area. The determination results of fundamental indicators of oily wastewater are as follows: Initial WCO concentration is 20 g/L, initial pH is 5.64 ± 0.04, initial OD600 is 0.312 ± 0.005, initial COD concentration is 12500.0 ± 707.1 mg/L, N-NO3− content is 56.44 ± 0.17 mg/L, and TP content is 80.16 ± 0.20 mg/L.
After 72 h of cultivation, the WCO degradation rate of the CK group was 3.67 ± 2.13%, and that of the SY group was 61.13 ± 7.30%. Confidently, the addition of bacterial combinations resulted in significant degradation of WCO. However, the intensity of degradation is decreasing (61.13 ± 7.30%) in oily wastewater, while in DM, it can be almost completely degraded (97.11 ± 2.22%). Oily wastewater did not contain as many nutrients as DM does. On the other hand, the pH was low and nearby microorganisms were competing for attention (Ke et al. 2021). These factors made it difficult for the additional strains to develop and reproduce normally and lessened their ability to degrade WCO. Compared to the bacterial communities isolated by Alexis et al. in wastewater samples, the proportion of oil in wastewater in this study is larger, and the separated and combined bacterial communities exhibit more efficient oil degradation rate (Nzila et al. 2017a). Gao et al. isolated three types of oil degrading bacteria, and the degradation rate of high concentration oil (6–8%, v/v) was 50–70% within 72 h (Gao et al. 2019). However, the strains were not optimized and were not applied to actual oily wastewater. The changes in the other indicators are shown in the Fig. 5. The pH of the CK group gradually rose to 6.95 ± 0.22 within 0–48 h and then became stable, reaching 6.85 ± 0.13 at 72 h, which may be due to the alkaline substances produced by the growth of indigenous strains, resulting in a pH increase. The pH of the SY group showed a downward trend within 0–48 h and dropped to 4.63 ± 0.06 at 48 h. There are two reasons for the pH decrease.
On the one hand, microorganisms degrade WCO into glycerol and fatty acids, and the accumulation of fatty acids reduces pH (Tang et al. 2012). On the other hand, lactic acid is the main metabolic end-product of carbohydrate fermentation (Zapasnik et al. 2022). OD600 reflects the change in the biomass of the strain and directly reflects the growth state of the microorganism. The evolution of OD600 is shown in the Fig. 5C, and the biomass change in the CK and SY groups was very similar within 0–24 h. However, the biomass of the SY group increased rapidly after 24 h, and at 48 h and 72 h, it was significantly higher than that of the CK group. This occurrence might be the result of the SY group's additional strains using WCO as a carbon source during mass reproduction. In contrast, the CK group used fewer WCO strains, resulting in differences in the biomass of the two groups, which preliminarily verified the WCO degradation ability of the added strains. A significant change in COD concentration was evident at 24 h, possibly due to the growth of indigenous microorganisms consuming more COD. The rate of COD consumption in the SY group accelerated from 24 to 72 h, and the growth rate determined at OD600 was also significantly accelerated, indicating that the added strains may have rapidly increased during this period. Finally, the COD degradation rate of the CK group was 55.60% ± 0.71%, and that of the SY group was 62.4% ± 5.65%. The reduction rate of TP concentration and N-NO3− concentration in the SY group was higher than CK group within 24 h to 72 h. The additional strains also promoted the degradation of TP and N-NO3−, which reduced the concentration of TP and N-NO3− to 8.23 ± 0.17 mg/L and 25.42 ± 1.63 mg/L, respectively.
Interaction between bacteria alliance and indigenous wastewater bacteria
To explore the difference between the bacterial alliance and the local bacterial community in oily wastewater, the bacterial communities of the experimental and control groups at 0 h and 72 h were measured (Fig. 6). Figure 6A shows that the number of taxa in the experimental and control groups increased between 0 and 72 h. The number of taxa in the control group was higher than that in the experimental group, indicating that the bacterial community was more abundant in the control group than in the experimental group. Figure 6B and Fig. 6C show that the quantities of YZQ-1 and YZQ-3 at 0 h were considerable. However, at 72 h, the amounts of YZQ-1 and YZQ-3 decreased relatively, indicating that YZQ-1 and YZQ-3 were adaptive in wastewater but only reached fourth and fifth places in abundance. The relative abundances of Weissella, Leuconostoc, and Lactococcus in the experimental group were higher than those in the control group at 72 h. Among these, Lactococcus showed the most significant change. The results showed that the competitiveness of Weissella, Leuconostoc, and Lactococcus in wastewater was enhanced after joining the bacterial alliance.
Relative abundance was among the top three in the experimental group. Weissella can ferment glucose, maltose, sucrose, and other sugars using hexose phosphate and phosphoketolase. It can produce lactic acid by heterotypic fermentation of glucose and maintain growth with carbohydrates as a fermentation substrate (Fusco et al. 2015). Leuconostoc and Lactococcus. are often used to ferment dairy products. They can ferment lactic acid (Masood et al. 2011). This increase was the reason for the lower pH in the experimental group. An instable pH environment inhibits bacterial alliances, leading to disadvantages. Like the composting results for food waste, lactic acid bacteria became the dominant genus in the system (Jeon et al. 2020). Therefore, developing a suitable aeration system in the bioreactor is necessary to provide a more sustainable treatment strategy. This can effectively avoid the local sterile area, and the inoculated microbial flora will maintain wastewater treatment for a longer time to maintain an adequate WCO reduction capacity. In addition, carriers can immobilise bacteria, reuse microbial cells, and serve as cell seed storage banks to maintain long-term environmental stability. Thus, we could develop an efficient bacterial alliance and a suitable vector (Es et al. 2015).
Conclusions
Through gradient adaptation, screening, and combination, this study established a highly efficient oil-degrading bacteria alliance composed of Bacillus tropicus, Pseudomonas multiresinivorans, and Raoultella terrigena. Successfully applied it to reduce the WCO in oily wastewater by 61.13 ± 7.30% and COD by 62.40 ± 5.65% within 72 h. YZQ-1 and YZQ-3 in the bacterial alliance were stable, whereas YZQ-4 did not survive for more than 72 h in this system. Lactic acid bacteria gradually became the dominant bacteria during the treatment process. The reason for the loss of the advantages of the bacterial alliance may be the low pH and low dissolved oxygen content of the environment. Therefore, further studies on combining efficient WCO degrading bacterial alliances and cell immobilisation are necessary. This combination can be a more efficient and sustainable strategy.
Data availability
Not applicable.
References
Affandi IE, Suratman NH, Abdullah S, Ahmad WA, Zakaria ZA (2014) Degradation of oil and grease from high-strength industrial effluents using locally isolated aerobic biosurfactant-producing bacteria. Int Biodeterior Biodegrad 95:33–40
Ahmad T, Belwal T, Li L, Ramola S, Aadil RM, Abdullah Xu YX, Luo ZS (2020) Utilization of wastewater from edible oil industry, turning waste into valuable products: A review. Trends Food Sci Technol 99:21–33
Awasthi MK, Selvam A, Chan MT, Wong JWC (2018) Bio-degradation of oily food waste employing thermophilic bacterial strains. Biores Technol 248:141–147
Cao X, Zhao Y (2009) The influence of sodium on biohydrogen production from food waste by anaerobic fermentation. J Mater Cycles Waste Manag 11(3):244–250
Chandra P, Enespa, Singh R, Arora PK (2020) Microbial lipases and their industrial applications: a comprehensive review. Microb Cell Factories 19(1)
Chandrasekhar SS, Sekhar SC, Kalyani S, Sridhar S (2022) Performance assessment of a side-stream membrane bioreactor for the treatment of kitchen wastewater. Biochem Eng J 180
Cheng SK, Zhao MY, Mang HP, Zhou XQ, Li ZF (2017) Development and application of biogas project for domestic sewage treatment in rural China: opportunities and challenges. J Water Sanit Hyg Dev 7(4):576–588
Datta P, Tiwari P, Pandey LM (2018) Isolation and characterization of biosurfactant producing and oil degrading Bacillus subtilis MG495086 from formation water of Assam oil reservoir and its suitability for enhanced oil recovery. Biores Technol 270:439–448
Es I, Vieira JDG, Amaral AC (2015) Principles, techniques, and applications of biocatalyst immobilization for industrial application. Appl Microbiol Biotechnol 99(5):2065–2082
Fusco V, Quero GM, Cho GS, Kabisch J, Meske D, Neve H, Bockelmann W, Franz C (2015) The genus Weissella: taxonomy, ecology and biotechnological potential. Front Microbiol 6
Gao L-L, Lu Y-C, Zhang J-L, Li J, Zhang J-D (2019) Biotreatment of restaurant wastewater with an oily high concentration by newly isolated bacteria from oily sludge. World J Microbiol Biotechnol 35(11):179
Jeon D, Chung K, Shin J, Park CM, Shin SG, Kim YM (2020) Reducing food waste in residential complexes using a pilot-scale on-site system. Bioresour Technol 311
Ke X, Sun JC, Liu C, Ying JM, Zou SP, Xue YP, Zheng YG (2021) Fed-in-situ biological reduction treatment of food waste via high-temperature-resistant oil degrading microbial consortium. Bioresour Technol 340
Ktata A, Krayem N, Aloulou A, Bezzine S, Sayari A, Chamkha M, Karray A (2020) Purification, biochemical and molecular study of lipase producing from a newly thermoalkaliphilic Aeribacillus pallidus for oily wastewater treatment. J Biochem 167(1):89–99
Le T-S, Nguyen P-D, Ngo HH, Bui X-T, Dang B-T, Diels L, Bui H-H, Nguyen M-T, Le Quang D-T (2022) Two-stage anaerobic membrane bioreactor for co-treatment of food waste and kitchen wastewater for biogas production and nutrients recovery. Chemosphere 309:136537
Li TY, Liu L, Wei XH, Zhang HY, Fang P (2014) Comparison of the food waste degradation rate and microbial community in the matrix between two biodegradation agents in a food waste disposer. Waste Manag 34(12):2641–2646
Mannu A, Poddighe M, Garroni S, Malfatti L (2022) Application of IR and UV–VIS spectroscopies and multivariate analysis for the classification of waste vegetable oils. Resour Conserv Recycl 178:106088
Masood MI, Qadir MI, Shirazi JH, Khan IU (2011) Beneficial effects of lactic acid bacteria on human beings. Crit Rev Microbiol 37(1):91–98
Matsuoka H, Miura A, Hori K (2009) Symbiotic effects of a lipase-secreting bacterium, Burkholderia arboris SL1B1, and a glycerol-assimilating yeast, Candida cylindracea SL1B2, on triacylglycerol degradation. J Biosci Bioeng 107(4):401–408
Matwijczuk A, Zając G, Karcz D, Chruściel E, Matwijczuk A, Kachel-Jakubowska M, Łapczyńska-Kordon B, Gagoś M (2018) Spectroscopic studies of the quality of WCO (Waste Cooking Oil) fatty acid methyl esters. Bio Web Conf 10
Mishra A, Tiwari P, Pandey LM (2023) Surface, interfacial and thermodynamic aspects of the Rhamnolipid-salt systems. J Mol Liq 384:122245
Mongkolthanaruk W, Dharmsthiti S (2002) Biodegradation of lipid-rich wastewater by a mixed bacterial consortium. Int Biodeterior Biodegrad 50(2):101–105
Montagnolli RN, Lopes PRM, Bidoia ED (2009) Applied models to biodegradation kinetics of lubricant and vegetable oils in wastewater. Int Biodeterior Biodegrad 63(3):297–305
Nimkande VD, Bafana A (2022) A review on the utility of microbial lipases in wastewater treatment. J Water Process Eng 46
Nzila A, Thukair A, Sankara S, Abdur Razzak S (2017a) Characterization of aerobic oil and grease-degrading bacteria in wastewater. Environ Technol 38(6):661–670
Nzila A, Thukair A, Sankara S, Razzak SA (2017b) Characterization of aerobic oil and grease-degrading bacteria in wastewater. Environ Technol 38(6):661–670
Pendashteh AR, Fakhru’l-Razi A, Chuah TG, Radiah ABD, Madaeni SS, Zurina ZA (2010) Biological treatment of produced water in a sequencing batch reactor by a consortium of isolated halophilic microorganisms. Environ Technol 31(11):1229–1239
Rajpal A, Arora S, Bhatia A, Kumar T, Bhargava R, Chopra AK, Kazmi AA (2014) Co-treatment of organic fraction of municipal solid and sewage by vermireactor waste (OFMSW). Ecol Eng 73:154–161
Ren J, Fan B, Huhetaoli, Niu D, Gu Y, Li C (2021) Biodegradation of Waste Cooking Oils by Klebsiella quasivariicola IUMR-B53 and Characteristics of Its Oil-Degrading Enzyme. Waste Biomass Valorization 12(3):1243–1252
Sarac N, Ugur A (2016) A green alternative for oily wastewater treatment: lipase from Acinetobacter haemolyticus NS02-30. Desalin Water Treat 57(42):19750–19759
Sharma S, Pandey LM (2020) Production of biosurfactant by Bacillus subtilis RSL-2 isolated from sludge and biosurfactant mediated degradation of oil. Biores Technol 307:123261
Sharma S, Datta P, Kumar B, Tiwari P, Pandey LM (2019) Production of novel rhamnolipids via biodegradation of waste cooking oil using Pseudomonas aeruginosa MTCC7815. Biodegradation 30(4):301–312
Sharma S, Verma R, Dhull S, Maiti SK, Pandey LM (2022) Biodegradation of waste cooking oil and simultaneous production of rhamnolipid biosurfactant by Pseudomonas aeruginosa P7815 in batch and fed-batch bioreactor. Bioprocess Biosyst Eng 45(2):309–319
Shi K, Zhou WZ, Zhao HX, Zhang YZ (2012) Performance of halophilic marine bacteria inocula on nutrient removal from hypersaline wastewater in an intermittently aerated biological filter. Biores Technol 113:280–287
Sutar VP, Mali GV, Upadhye V, Singh VK, Sinha RP (2023) Purification of Lipase from Pseudomonas aeruginosa VSJK R-9 and Its Application in Combination with the Lipolytic Consortium for Bioremediation of Restaurant Wastewater. Appl Biochem Biotechnol 195(3):1888–1903
Szymczak T, Cybulska J, Podlesny M, Frac M (2021) Various Perspectives on Microbial Lipase Production Using Agri-Food Waste and Renewable Products. Agriculture-Basel 11(6)
Tang HL, Xie YF, Chen Y-C (2012) Use of Bio-Amp, a commercial bio-additive for the treatment of grease trap wastewater containing fat, oil, and grease. Biores Technol 124:52–58
Tong YJ, Zuo CJ, Ding WL, Jiang SH, Li WX, Xing WH (2021) Sulfonic nanohydrogelled surface-modified microporous polyvinylidene fluoride membrane with excellent antifouling performance for treating water-oil separation of kitchen wastewater. J Membr Sci 628
Wang TZ, Wang WJ, Hu HY, Khu ST (2022) Novel Quantitative Evaluation of Biotreatment Suitability of Wastewater. Water 14(7)
Xing M, JianYang, Wang Y, Liu J, Yu F (2011) A comparative study of synchronous treatment of sewage and sludge by two vermifiltrations using an epigeic earthworm Eisenia fetida. J Hazard Mater 185(2):881–888
Xu S, Tao LD, Wang JJ, Zhang XX, Huang ZY (2022) Rapid in-situ aerobic biodegradation of high salt and oily food waste employing constructed synthetic microbiome. Eng Life Sci 1–15
Yang J, Zhao Z-Q, Wang M, Yu K-F, Zhang T, Lin H, Zheng H-B (2022) Biodegradation of tylosin in swine wastewater by Providencia stuartii TYL-Y13: Performance, pathway, genetic background, and risk assessment. J Hazard Mater 440:129716
Yang J, Xu S-Y, Zhang T, Zhao Z-Q, Xie X-J, Wang W-F, Zhang C, Zheng H-B (2023) A dual bacterial alliance removed erythromycin residues by immobilizing on activated carbon. Biores Technol 384:129288
Yoo H-Y, Simkhada JR, Cho SS, Park DH, Kim SW, Seong CN, Yoo JC (2011) A novel alkaline lipase from Ralstonia with potential application in biodiesel production. Biores Technol 102(10):6104–6111
Zahri KN, Zulkharnain A, Gomez-Fuentes C, Sabri S, Abdul Khalil K, Convey P, Ahmad SA (2021a) The Use of Response Surface Methodology as a Statistical Tool for the Optimisation of Waste and Pure Canola Oil Biodegradation by Antarctic Soil Bacteria. in: Life 11
Zahri KN, Zulkharnain A, Sabri S, Gomez-Fuentes C, Ahmad SA (2021b) Research Trends of Biodegradation of Cooking Oil in Antarctica from 2001 to 2021: A Bibliometric Analysis Based on the Scopus Database. In: Int J Environ Res Public Health 18
Zapasnik A, Sokolowska B, Bryla M (2022) Role of Lactic Acid Bacteria in Food Preservation and Safety. Foods 11(9)
Zhou SP, Zhou HY, Xia SN, Ying JM, Ke X, Zou SP, Xue YP, Zheng YG (2021) Efficient bio-degradation of food waste through improving the microbial community compositions by newly isolated Bacillus strains. Bioresour Technol 321
Zou XL (2015) Treatment of heavy oil wastewater by UASB-BAFs using the combination of yeast and bacteria. Environ Technol 36(18):2381–2389
Funding
This research was supported by the Zhejiang Province Key Research and Development Plan (2021C03190), the Zhejiang Province "Sannong Jiufang" (2022SNJF077), and the 111 Project (D18008).
Author information
Authors and Affiliations
Contributions
All authors contributed to the study conception and design. Material preparation were performed by Heng-yuan Chen and Wen-fan Wang. Data collection were performed by Xiao-jian Lian and Xiao-jie Xie. Data analysis were performed by Zhuo-qun Zhao and Jian Yang. The design of experiment were performed by Min Wang, Ke-fei Yu and Hua-bao Zheng. The first draft of the manuscript was written by Zhuo-qun Zhao and all authors commented on previous versions of the manuscript. All authors read and approved the final manuscript.
Corresponding author
Ethics declarations
Ethical approval and consent to participate
Not applicable.
Consent for publication
Not applicable.
Competing interests
The authors declare that they have no competing interests.
Additional information
Responsible Editor: Robert Duran
Publisher's Note
Springer Nature remains neutral with regard to jurisdictional claims in published maps and institutional affiliations.
Supplementary Information
Below is the link to the electronic supplementary material.
Rights and permissions
Springer Nature or its licensor (e.g. a society or other partner) holds exclusive rights to this article under a publishing agreement with the author(s) or other rightsholder(s); author self-archiving of the accepted manuscript version of this article is solely governed by the terms of such publishing agreement and applicable law.
About this article
Cite this article
Zhao, Zq., Yang, J., Chen, Hy. et al. Construction and application of highly efficient waste cooking oil degrading bacteria consortium in oily wastewater. Environ Sci Pollut Res 30, 125677–125688 (2023). https://doi.org/10.1007/s11356-023-31107-1
Received:
Accepted:
Published:
Issue Date:
DOI: https://doi.org/10.1007/s11356-023-31107-1