Abstract
The complexes formed from uranyl salts and tri-n-butyl phosphate (TBP) in ionic liquids (ILs) were studied by spectroscopic methods. [UO2(TBP)4]2+ is formed from UO2(ClO4)2·xH2O with excess of TBP in ILs. The coordination number of uranyl in [UO2(TBP)4]2+ is determined as 4 by ATR–FTIR study. In [Bmim][NTf2], though TBP cannot replace the NO3 − coordinated to uranyl, TBP/[Bmim][NTf2] can extract “nitrate-free” uranyl complex from diluted HNO3 medium and the extracted complex is converted into [UO2(TBP)4]2+ after drying. The formation of [UO2(TBP)4]2+ provides spectroscopic evidence for the cation-exchange mechanism of uranyl extraction by TBP/[Bmim][NTf2] from diluted HNO3 medium.
Similar content being viewed by others
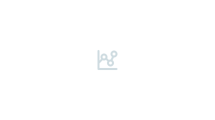
Avoid common mistakes on your manuscript.
Introduction
Ionic liquids (ILs) are molten salts with low melting points below 373 K, consisted of large organic cations and anions. For their ionic nature, ILs have some unique advantages over common molecular organic solvents, e.g. low vapor pressure, good conductivity, and solubility for inorganic compounds, thus have been extensively studied for their applications in many fields such as separation, synthesis, catalysis, and electrochemistry [1–5]. The potentiality of ILs in nuclear chemistry, especially chemistry for spent nuclear fuel reprocessing, has also been explored in recent years. ILs can serve as alternatives either for volatile and flammable solvents such as kerosene used in the commercial plutonium uranium reduction extraction (PUREX) process or for corrosive high temperature inorganic molten salts in the pyrochemical process [6–11].
ILs with hexafluorophosphate ([PF6]−) and bis(trifluoromethanesulfonyl)imide ([(CF3SO2)2N]− or [NTf2]−) as anions are generally hydrophobic, thus regarded as potential alternative solvents in extraction studies [3, 7, 9, 10]. The [NTf2]− based ILs are becoming popular, for their low viscosity, resistance to hydrolysis, and better thermo-stability [12]. Ion exchange extraction is not favorable in molecular solvents but common in ILs, and benefiting from this unique mechanism, a number of metal ions are extracted into ILs with enhancing efficiency [3, 7, 9, 10]. The loss of ILs components is a major disadvantage of ion-exchange extraction [7, 9, 10], however, this disadvantage could be economically compensated by the large improvement of extraction efficiency in many cases, especially for separation and recovery of precious or hazardous materials. As the extract agent for PUREX process, tri-n-butyl phosphate (TBP) is of great importance in the extraction chemistry of actinides, a number of studies involving extraction of uranium into ILs also use TBP [13–17]. Giridhar et al. [13] investigated the extraction of uranyl (UO2 2+) by TBP in 1-butyl-3-methylimidazolium hexafluorophosphate ([Bmim][PF6]) from aqueous HNO3 solutions, finding that the extraction behavior of TBP in [Bmim][PF6] is similar with that in n-dodecane and the extracted complex is [UO2(NO3)2(TBP)2]. On the hand, the extraction of uranyl by TBP in [NTf2]− based ILs seems more complicated. Several groups have studied the extraction of uranyl by TBP in [NTf2]− based ILs with imidazolium [14–16] or quaternary ammonium [17] cations. The U-shaped distribution rate (DU) curve is thought to be result of change in the extraction mechanism, from cation exchange at low c(HNO3) to either neutral extraction or anion exchange [9, 10]. The high DU for TBP in [NTf2]− based ILs at low aqueous c(HNO3) is much different from the case of the conventional extraction in molecular solvents. Though the extraction of uranyl by TBP/ILs was extensively investigated, studies on interactions between uranyl and TBP in ILs have rarely been reported.
Knowledge on the solvation and complexation of metal ions in ILs is very important to understand the extraction process. Uranyl complexes often show characteristic vibronic fine structures in their optical (UV–Vis absorption and fluorescence emission) spectra, and those spectral details are mainly determined by the symmetry of the first coordination sphere of uranyl, while less affected by the chemical nature of the ligands [18–21]. Hence optical spectroscopic methods are often used to study the coordination chemistry of uranyl in solution. There are a number of literatures on optical spectra of uranyl complexes in ILs, mainly involving inorganic ligands such as Cl− and NO3 −, thus [UO2Cl4]2− and [UO2(NO3)3]- become the most well-studied [22–28]. Vibrational spectroscopy is also important in studies of uranyl complexes. As an intact linear group, the uranyl has some characteristic vibrational modes, such as the Raman active symmetric stretching (ν s(UO2)) and the infrared (IR) active asymmetric stretching (ν as(UO2)). The frequencies of these modes are sensitive to the uranyl coordination environment, and thus can be used to investigate the interaction between ligands and uranyl [29, 30]. By taking advantage of its convenience in sample preparation and measurement [31, 32], attenuated total reflection-Fourier transform infrared (ATR–FTIR) spectrometry has been extensively used for coordination chemistry of uranyl in aqueous solutions [33–35] and ILs [36, 37]. Nonetheless, for complexes formed from uranyl and organic ligands such as TBP in ILs, few studies have been reported.
In this study, to understand the interactions between uranyl and TBP in ILs, we investigated the complexes formed from TBP with uranyl perchlorate (as a source of weak-coordinated uranyl) and uranyl nitrate in ILs, as well as the complex(es) extracted by TBP/IL via cation-exchange from diluted HNO3, by spectroscopic studies. The ILs used here are 1-butyl-3-methylimidazolium bis(trifluoromethanesulfonyl)imide ([Bmim] [NTf2]), N-n-butyl-N,N,N-trimethylammonium bis(trifluoromethanesulfonyl)imide ([N4111] [NTf2]), and N-methyl-N-butylpyrrolidinium bis(trifluoromethanesulfonyl)imide ([Pyr14] [NTf2]) (Fig. 1 illustrates the structures of the ions of these ILs).
Experimental
Materials
TBP (97 %) was purchased from Alfa-Aesar (Tianjin, China). Other reagents of A.R. grade were purchased from Sinopharm Chemical Reagent Company (Beijing, China), except for materials otherwise noted.
Ionic liquids
[Bmim][NTf2] was prepared by reaction of aqueous solutions of [Bmim]Cl (99 %, Lanzhou Institute of Chemical Physics, CAS, Lanzhou, China) and Li[NTf2] (99 %, TCI, Japan), according to the procedure described in Ref. [12]. [N4111][NTf2] and [Pyr14][NTf2] were prepared in the same manner as [Bmim][NTf2], whereas the starting material [Bmim]Cl were replaced by [N4111]Br and [Pyr14]Br (both from Lanzhou Institute of Chemical Physics with 99 % purity). The ILs were washed with deionized water for several times until no Cl− or Br− was detected in the aqueous phase by AgNO3 (For Cl− detection in aqueous solutions, the detection limit of AgNO3 method is less than 10 ppm [38]). After drying under vacuum at 343 K for >12 h, the water amount was found below 100 ppm as measured by Karl Fischer titration.
Sample preparation
UO2(ClO4)2·xH2O was previously prepared in our lab [39] by dissolving UO3 with perchloric acid according to literatures [28, 40]. The resulting yellow solid was dissolved in ILs to prepare stock solutions with uranyl concentration c(UO2 2+) ~0.2 M, and the stock solutions were stored in dark in a desiccator. (Caution Heating a mixture of perchloric acid or its salt solution with an organic material to dryness may cause explosion!)
The samples for spectroscopic measurement were prepared by mixing the UO2(ClO4)2·xH2O stock solutions with adequate amount of TBP (by volume of TBP or TBP/ILs stock solutions) and then diluting with the corresponding ILs, and following vigorously shaking with a vortex mixer. Samples using UO2(NO3)2 were also prepared in this manner with UO2(NO3)2·6H2O as uranyl source.
For the extracted samples, aqueous phase (UO2(NO3)2 in 0.01 M HNO3) and organic phase (1 M TBP/[Bmim][NTf2]) were added to a centrifuge tube with a 1:1 volume ratio (1.6 mL in total), and then vigorously shaken with a vortex mixer for 2 min, followed by centrifugation at 3000 rpm for 10 min. The initial aqueous UO2(NO3)2 concentrations are 0.01 M for UV–Vis and 0.05 M for ATR–FTIR, and 80 % of uranyl was extracted into organic phases according to the UV–Vis spectra of aqueous phases. The resulting organic phase was then dried under vacuum at 343 K for >12 h, if necessary.
Instrumental methods
The UV–Vis spectra were recorded in 340-530 nm region with a Shimadzu UV-2450 spectrophotometer (Shimadzu Corporation, Kyoto, Japan) using 1 cm quartz cells. Emission spectra were recorded with a Hitachi F4500 fluorescence spectrometer (Hitachi High-Technologies Corporation, Tokyo, Japan) with a 150 W xenon lamp. The excitation wavelength (λexc) was 330 nm (The time for a single scan is less than 5 min, and the UV–Vis spectra of samples before and after fluorescence measurement show no visible change). The infrared spectra were recorded with a Shimadzu IR Affinity-1 infrared spectrometer (Shimadzu Corporation, Kyoto, Japan) equipped with a PIKE horizontal attenuated total reflectance (HATR) accessory (PIKE Technologies, Madison, WI, USA). A 45° Ge through plate was used as a reflection medium. The spectra in 800–4000 cm−1 region were acquired with 20 times scans at resolution of 2 cm−1.
Results and discussion
UV–Vis spectra and fluorescence emission spectra of [UO2(TBP)4]2+
The UV–Vis spectra of 0.010 M UO2(ClO4)2·xH2O with 0.10 M TBP in three ILs ([Bmim][NTf2], [N4111][NTf2], and [Pyr14][NTf2]) are showing in Fig. 2, as well as the spectra of UO2(ClO4)2·xH2O in [Bmim][NTf2] and in pure TBP. [UO2(TBP)4]2+ is formed when dissolving UO2(ClO4)2 in TBP [41]. Since [NTf −2 ] is a weakly coordinating anion to uranyl [42], it is speculated that similar complex can also be formed by adding excess of TBP into [NTf −2 ]-based ILs containing UO2(ClO4)2. The spectra of uranyl-TBP complexes in the ILs (Fig. 2b, c, and d) have almost identical characters, so the structures of those complexes should be same. And those spectra are similar with the spectrum of [UO2(TBP)4]2+ in TBP (Fig. 2e), suggesting that the uranyl-TBP complex in the ILs could also be [UO2(TBP)4]2+. The minor differences in peak wavelength (<1 nm) and band shape can be attributed to solvent effects. In contrast, the spectrum of UO2(ClO4)2·xH2O without TBP (Fig. 2a) is much different.
In spectra of uranyl complexes, the characteristic vibronic fine structure is mainly determined by the certain symmetry (and geometry) of the first coordination sphere in the uranyl equatorial plane [18–21]. Thus the structure of [UO2(TBP)4]2+ can also be supported by its spectral similarity with other known uranyl complexes with tetragonal coordination in the equatorial plane. [UO2Cl4]2− is a typical complex with D4h symmetry, and its UV–Vis spectra in non-aqueous molecular solvents and ILs have been well studied [22–24, 28, 40]. The UV–Vis spectrum of [UO2(TBP)4]2+ is similar with that of [UO2Cl4]2− (Fig. S1 in SI), suggesting their similarity in symmetry. Moreover, our group have reported the dicationic complex formed in ILs by uranyl and a bidentate chelate, tetraethyl methylenebisphosphonate (TEMBP), with the composition of [UO2(TEMBP)2]2+ [39]. Both having tetragonal coordination by P=O ligands, the spectra of [UO2(TBP)4]2+ and [UO2(TEMBP)2]2+ in ILs are similar, with the peak maxima difference <1 nm (for data of UV–Vis spectra of [UO2(TBP)4]2+, see Table S1 in SI). The proposed symmetry of [UO2(TBP)4]2+ is D4h, and its spectrum can be interpreted preliminarily according to the spectral similarity with that of [UO2Cl4]2−. The spectra can be separated into two regions [22]: the region I represents the bands in the range from 22,000 to 25,000 cm−1 (400–454 nm in wavelength) for transitions \(\varSigma g^{ + } \to \varPhi g{\text{ and }}\varSigma g^{ + } \to \varDelta g\); and region II represents the bands in the range below 22,000 cm−1 (>454 nm in wavelength) for transitions \(\varSigma g^{ + } \to \varPi g{\text{ and }}\varSigma g^{ + } \to \varDelta g\). In region II, [UO2(TBP)4]2+ shows three intense bands at 495, 478 and 462 nm, while similar bands of [UO2Cl4]2− are at 492, 475 and 459 nm. These bands belong to a vibronic progression with peak separation of about 720 cm−1, attributed to the O=U=O symmetric stretching (ν s) frequency in the first excited state (the predominant vibrational mode coupling with the absorption spectrum) [18–21].
In [UO2(TBP)4]2+, though the coordination number of TBP is 4, uranyl cannot be fully coordinated by TBP to form this complex with stoichiometric ligands, as evidenced by spectrum Fig. 3a. The spectrum with ligand-to-uranyl (L/U) ratio of 4 has sharp and intense bands in region I and broad and weak bands in region II, suggesting that the corresponding uranyl complex may not be [UO2(TBP)4]2+, and the sharp bands could come from an intermediate complex with mixed coordination by TBP and H2O (or [NTf2]− and/or [ClO4]−). With increasing L/U ratios, the spectra change drastically for the shrink of bands in region I and growth in region II (Fig. 3b, c). No visible spectral change can be observed when the L/U ratio exceeds 8 (Fig. 3c, d), indicating the complete coordination of uranyl by TBP. The gradual change of spectra of L/U ratio from 4 to 8 demonstrates the move of equilibrium:
This fact suggests that TBP is not a “very strong” ligand for uranyl in ILs. By contrast, uranyl is completely coordinated to form [UO2Cl4]2− with stoichiometric [24] or slightly excess (5 eq.) [28] of Cl−.
Figure 4 illustrates the emission spectra of 0.01 M UO2(ClO4)2·xH2O with variety amount of TBP in [N4111][NTf2] in the wavelength range from 470 to 580 nm. A remarkable enhancement of fluorescence intensity is observed as the L/U ratio increases from 4 to 8. For samples of L/U > 8, the difference in peak intensities of spectra is unremarkable (<5 %) (See Fig. S2 in SI). This trend of spectral change with increasing amount of TBP is the same as that of the corresponding absorption spectra. Interestingly, though the absorption is weaker, the emission intensity of [UO2(TBP)4]2+ is much higher than those of the intermediate species.
The emission spectrum of [UO2(TBP)4]2+ is predominantly coupled with the symmetrical stretching frequency (ν as) of uranyl in the ground state (~850 cm−1), characterized by the peaks at 497, 519, 543 and 569 nm (20,105, 19,260, 18,416 and 17,575 cm−1), which are corresponded to the typical peaks of the electronic transition \({\text{S}}_{ 10} \to {\text{S}}_{0v}\) [18–21]. No obvious shift of the peaks can be observed with the variation of TBP concentration, meaning no distinct structure change. As shown in the insert graph of Fig. 4, the emission spectrum shows a typical mirror-relationship with the corresponding absorption spectrum in region II. The common band at 495 (absorption)/497 (emission) is identified as the first electronic transition (the 0–0 transition), and this transition is predominantly coupled with the symmetrical stretching frequency of uranyl as discussed above. The emission spectra of [UO2(TBP)4]2+ and [UO2(TEMBP)2]2+ [39] are almost the same in shape, as well as the mirror-relationship with their absorption spectra, suggesting their structural similarity.
ATR–FTIR spectra of [UO2(TBP)4]2+
Though uranyl cannot be completely coordinated by TBP until L/U = 8 in the UV–Vis study with c(uranyl) = 0.01 M, at a higher concentration level such as 0.1 M uranyl, 0.5 M TBP is enough to complete the coordination as evidenced by UV–Vis spectrum(data not shown). It is because that in the equilibrium of Eq. (1), the ratio of [UO2(TBP)4]2+/[UO2(TBP)4−x ]2+ is controlled by residual TBP concentration, and which is 0.04 M in the former case and 0.10 M in the later. Since the ν as(O=U=O) band in ATR–FTIR spectra is less sensitive to uranyl concentration, higher uranyl concentrations up to 0.1 M were used in the ATR–FTIR study.
Figure 5 shows the ν as(O=U=O) region of ATR–FTIR spectra of 0.1 M UO2(ClO4)2·xH2O (a), 0.1 M [UO2(TBP)4]2+ (c), and 0.5 M TBP (b) in [Bmim][NTf2]. For UO2(ClO4)2·xH2O, the ν as(O=U=O) band appears at 968 cm−1, indicating the weak coordination environment of uranyl [36, 37]. When coordinated by 4 TBP ligands, the ν as(O=U=O) band of [UO2(TBP)4]2+ shifts to 940 cm−1. The bands at 960 cm−1 and high wavenumbers in spectra b and c are due to the TBP ligand.
TBP has a strong ν(P=O) absorption band at ~1280 cm−1, which shifts to lower wavenumber when coordinated to metal ions. This feature was used to determine the coordination number of TBP to UO2(ClO4)2·xH2O in molecular solvent [41]. Fortunately, this band is located in the “plain region” of 1240–1320 cm−1 in the IR spectrum of [Bmim][NTf2] (thus the IL background affects little on the measurement of ν(P=O) band), though the peak shifts to 1261 cm−1 due to solvent effect (Fig. S3 in SI). For spectra of [Bmim][NTf2] solutions of TBP, by subtraction a straight baseline from 1242 to 1290 cm−1, the absorbance at 1261 cm−1 shows good linear relationship versus TBP concentration with R 2 = 0.9997 (Fig. S4 in SI). To determine the coordination number of [UO2(TBP)4]2+ in [Bmim][NTf2], 0.02–0.10 M UO2(ClO4)2·xH2O was added to solutions containing 0.50 M TBP, and then scanned their ATR–FTIR spectra. The differential spectra of uranyl containing samples against 0.50 M TBP in [Bmim][NTf2] are showing in Fig. 6. The concentrations of residual free TBP c(TBP) were calculated according to the absorbance at 1261 cm−1. As shown in the insert graph of Fig. 6, c(TBP) shows a linear relationship versus uranyl concentration c(uranyl) with a slope of −4.12 ± 0.04, indicating that there are 4 TBP ligand coordinating to uranyl in [UO2(TBP)4]2+. That is a direct evidence on the coordination number in [UO2(TBP)4]2+.
Additional information on the interaction of TBP and UO2(ClO4)2·xH2O in [Bmim][NTf2] can also be obtained. The band at 1172 cm−1 is due to ν(P=O) of coordinated TBP, and its intensity increases with the addition of UO2(ClO4)2·xH2O, as well as the ν as(O=U=O) band of [UO2(TBP)4]2+ at 940 cm−1 and the ν 3 band of free ClO4 − at 1097 cm−1 [43]. Coordination to uranyl also affects some vibrational modes of the [PO4] group in TBP, as indicated by the sharp increase at 1042 cm−1 and decrease at 992 cm−1, and similar intensity changes in TBP-uranyl nitrate system were reported by K. Nukada et al. (increase at 1058 cm−1 and decrease at 998 cm−1) [44]. The absorbance intensities of the bands mentioned above are all changed linearly according to uranyl concentration, as showing in Fig. S5 in SI.
Uranyl nitrate with TBP in ILs
Complex formed by TBP with UO2(NO3)2 in [Bmim][NTf2] is also studied. Figure 7 shows the UV–Vis spectra of 0.1 M UO2(NO3)2 in pure TBP (a) and in [Bmim][NTf2] with 1 M TBP (b). It is well-known that UO2(NO3)2(TBP)2 is formed when dissolving UO2(NO3)2 in pure TBP or TBP-containing molecular solvents such as kerosene [30, 41]. The two spectra in Fig. 7 have same spectral fine structures in the 430–500 nm region, with the corresponding peak maxima difference <1 nm, suggesting the structure similarity of the complexes. However, their spectra in the 380–430 nm region are different, which could be the result of solvent effects (and the formation of UO2(NO3)2(TBP)2 is further confirmed by ATR–FTIR spectra in Fig. 8). The absorption data of spectra in Fig. 7 are listed in Table S2 in SI.
The formation of UO2(NO3)2(TBP)2 in 1 M TBP/[Bmim][NTf2] is also supported by results from ATR–FTIR study. As showing in Fig. 8 (left), the ν as(O=U=O) of UO2(NO3)2(TBP)2 in [Bmim][NTf2] is 941 cm−1 (a), very close to the value in pure TBP (b, 940 cm−1). This band shows a red-shift of 10 versus 951 cm−1 of UO2(NO3)2 in [Bmim][NTf2] [36, 37], as a result of TBP bonding to uranyl. The band in the ν(NO) region (centered at ~1530 cm−1) is evidence of NO3 − bonding to uranyl in a bidentate mode [29, 36, 37, 43]. The band centered at 1524 cm−1 of UO2(NO3)2(TBP)2 in [Bmim][NTf2] (spectra b in the right graph) has comparable intensity versus that of UO2(NO3)2 (band at 1535 cm−1 of spectra a), suggesting that two NO3 − ions are coordinating to uranyl in bidentate mode in the former complex. And the band position is also close to that of UO2(NO3)2(TBP)2 in pure TBP (1525 cm−1, spectra c), the red-shift of 11 cm−1 may due to change of uranyl-nitrate bonding strength which is effected by TBP-bonding. The ν as(O=U=O) and ν(NO) bands of UO2(NO3)2(TBP)2 in pure TBP are sharper than their counterparts in [Bmim][NTf2], it could be the result of different complex-solvent interactions.
The ν(P=O) band of free TBP is overlapped by the ν as(NO2) band (centered at ~1290 cm−1) [29, 43] of bonded NO3 − (Fig. S6 in SI), so the coordination number of TBP to UO2(NO3)2 cannot be determined by simply measuring the absorbance at 1261 cm−1. However, as discussed in the previous section, the bands at 992 and 1042 cm−1 are also changed linearly with increasing uranyl concentration, thus the intensities of these bands can be used to estimate the TBP coordination number when comparing with spectra of UO2(ClO4)2-TBP system as showing in Fig. S6 in SI. Given 4 TBP ligands coordinating to UO2(ClO4)2·xH2O in [Bmim][NTf2], the coordination number to UO2(NO3)2 is estimated to be 1.89 according to the 992 cm−1 negative band or 1.77 for the 1042 cm−1 band, agree with the formula of UO2(NO3)2(TBP)2. NO3 − is a strong ligand to uranyl in ILs [25–28], and the formation of UO2(NO3)2(TBP)2 in presence of excessive TBP in [Bmim][NTf2] indicates that TBP cannot replace the coordinating NO3 −.
Spectra of extracted uranyl-TBP complex
Extraction of uranyl from aqueous HNO3 solutions by TBP/ILs has been studied by several groups [13–17]. The extraction mechanism is regarded as a mixture of cation exchange and neutral extraction (or anion exchange) [9, 10]. In cases of low HNO3 concentration (e.g. 0.01 M), the extraction is dominated by cation exchange mechanism, and the extracted species is thought to be [UO2(TBP) x ]2+ without NO3 − (though the value of x is not the same for different literatures) [14–17]. If NO3 − does not participate in the extraction of uranyl, the extracted [UO2(TBP) x ]2+ complex should be either [UO2(TBP)4]2+ or a complex with mixed coordination of TBP and H2O.
Figure 9a is the UV–Vis spectrum of uranyl extracted by 1 M TBP/[Bmim][NTf2] from 0.01 M UO2(NO3)2/0.01 M HNO3, its spectral characters are different from [UO2(TBP)4]2+ in [Bmim][NTf2] (d) and similar with the extracted complex in TBP/ammonium IL (from 0.01 M HNO3) reported by Bell et al. [17]. Since the solubility of water in [Bmim][NTf2] is about 0.8 M (~1.4 % in weight) [45], the extracted complex could be a complex with mixed-coordination by TBP and water, [UO2(TBP) x (H2O) y ]2+. Figure 9b is the spectrum of 0.01 M UO2(ClO4)2·xH2O in water-saturated 1 M TBP/[Bmim][NTf2], it has almost the same spectral characters with spectrum a, and after drying of the extracted sample (c), it is converted to the same as the spectrum of [UO2(TBP)4]2+. Thus it is speculated that the uranyl complex extracted by 1 M TBP/[Bmim][NTf2] from 0.01 M HNO3 should be a nitrate-free [UO2(TBP) x (H2O) y ]2+ complex with mixed-coordination by TBP and water.
The extraction of nitrate-free [UO2(TBP) x (H2O) y ]2+ complex is also confirmed by ATR–FTIR spectra. Figure 10 and 11 show the IR spectra of the extracted complex (from 0.05 M UO2(NO3)2/0.01 M HNO3, with 80 % of uranyl extracted) in the ν as(O=U=O) and ν(NO) regions. The ν as(O=U=O) band in the extracted complex is at 943 cm−1 (Fig. 10a), with a blue-shift of 3 cm−1 comparing to that of [UO2(TBP)4]2+ (940 cm−1) in dry [Bmim][NTf2], suggesting that uranyl in this complex is in a mixed coordination environment from TBP and a weaker ligand (in this case, mostly be H2O). The controlled sample (Fig. 10b) with no uranyl extraction shows a plain background in 900–960 cm−1 region, and the negative band at 970–990 cm−1 could be the result of hydrogen-bond interactions between dissolved H2O and TBP.
In the nitrate stretching region (Fig. 11), both the extracted complex (a) and the controlled sample (b) show no visible band of ν(NO), comparing to the spectrum of [UO2(NO3)2(TBP)2] (c). Since IR spectrum of neat [Bmim][NTf2] shows two mild bands at 1573 and 1465 cm−1 (1575 and 1469 cm−1 in ref [46]), the small negative bands at the corresponding positions (Fig. 11a) are attributed to decrease of [Bmim]+ concentration, and loss of [Bmim]+ is the result of cation-exchange in the extraction progress. By analysis of the IR spectra in Fig. 11, it is confirmed that NO3 − is not involved in the extracted complex.
Though the NO3 − coordinating to uranyl cannot be replaced by excess TBP in [Bmim][NTf2], TBP/[Bmim][NTf2] can extract nitrate-free uranyl complex from aqueous media with low HNO3 concentrations. Because nitrate ion is a hydrophilic inorganic anion, in liquid–liquid extraction, it tends to retain in the aqueous phase, thus its concentration in IL phase could be very low.
Results from UV–Vis and ATR–FTIR studies on the extracted uranyl complex provide direct spectroscopic supports to the cation-exchange mechanism of uranyl extraction by TBP/[Bmim][NTf2] from low HNO3 concentration media, and this will be helpful to understand the complicated extraction mechanism in ILs.
Conclusions
The complexes formed from uranyl salts and TBP in [NTf2]− based ILs ([Bmim][NTf2], [N4111][NTf2] and [Pyr14][NTf2]) were studied by spectroscopic methods.
[UO2(TBP)4]2+ is formed from UO2(ClO4)2·xH2O with excess of TBP in [NTf2]− based ILs, evidenced by its UV–Vis absorption spectra. The UV–Vis spectrum shows typical vibronic fine structures of tetragonal coordination in the uranyl equatorial plane. Since TBP is not a very strong ligand, excess of ligand is necessary for complete coordination of uranyl. The fluorescence emission spectrum of [UO2(TBP)4]2+ also shows characteristic fine structures. The ν as(O=U=O) band of ATR–FTIR spectrum of [UO2(TBP)4]2+ in [Bmim][NTf2] shifts to 940 cm−1, from the 968 cm−1 band of UO2(ClO4)2·xH2O, showing the coordination effect. By quantitative study using the ν(P=O) band of free TBP ligand at 1261 cm−1, c(TBP) (for free ligand) is determined to be linearly changed with c(uranyl) and the slope is −4.12 ± 0.04, thus confirmed the composition of [UO2(TBP)4]2+.
Complex formed from UO2(NO3)2 with excess of TBP in [Bmim][NTf2] is UO2(NO3)2(TBP)2. The UV–Vis spectrum of UO2(NO3)2 in 1 M TBP/[Bmim][NTf2] is similar with that of UO2(NO3)2(TBP)2 in pure TBP. The band centered at 1524 cm−1 in its ATR–FTIR spectrum has comparable intensity versus that of UO2(NO3)2, indicating the retaining of two coordinating NO3 −. The formation of UO2(NO3)2(TBP)2 in presence of excessive TBP in [Bmim][NTf2] shows that TBP cannot replace the coordinating NO3 −. However, TBP/[Bmim][NTf2] can extract “nitrate-free” uranyl complex [UO2(TBP)x (H2O) y ]2+ from diluted HNO3 medium, this complex is converted into [UO2(TBP)4]2+ after drying as evidenced by UV–Vis spectra, and it is also confirmed that NO3 − is not involved in the extracted complex by ATR–FTIR.
The studies on [UO2(TBP)4]2+ and extracted [UO2(TBP) x (H2O) y ]2+ complex provide direct spectroscopic supports to the cation-exchange mechanism of uranyl extraction by TBP/[Bmim][NTf2] from low HNO3 concentration media, and this will be helpful to understand the complicated extraction mechanism in ILs.
References
Welton T (1999) Room-temperature ionic liquids. Solvents for synthesis and catalysis. Chem Rev 99:2071–2084
Wasserscheid P, Welton T (2002) Ionic liquids in synthesis. Wiley-VCH, Weinheim
Han X, Armstrong DW (2007) Ionic liquids in separations. Acc Chem Res 40:1079–1086
Hapiot P, Lagrost C (2008) Electrochemical reactivity in room-temperature ionic liquids. Chem Rev 108:2238–2264
Clare B, Sirwardana A, MacFarlane DR (2010) Synthesis, purification and characterization of ionic liquids. Top Curr Chem 290:1–40
Cocalia VA, Gutowski KE, Rogers RD (2006) The coordination chemistry of actinides in ionic liquids: a review of experiment and simulation. Coord Chem Rev 250:755–764
Binnemans K (2007) Lanthanides and actinides in ionic liquids. Chem Rev 107:2592–2614
Mudring AV, Tang S (2010) Ionic liquids for lanthanide and actinide chemistry. Eur J Inorg Chem 18:2569–2581
Billard I, Ouadi A, Gaillard C (2011) Liquid–liquid extraction of actinides, lanthanides, and fission products by use of ionic liquids: from discovery to understanding. Anal Bioanal Chem 400:1555–1566
Sun X, Luo H, Dai S (2011) Ionic liquids-based extraction: a promising strategy for the advanced nuclear fuel cycle. Chem Rev 112:2100–2128
Takao K, Bell TJ, Ikeda Y (2013) Actinide chemistry in ionic liquids. Inorg Chem 52:3459–3472
Bonhote P, Dias AP, Papageorgiou N, Kalyanasundaram K, Grätzel M (1996) Hydrophobic, highly conductive ambient-temperature molten salts. Inorg Chem 35:1168–1178
Giridhar P, Venkatesan KA, Srinivasan TG, Vasudeva Rao PR (2005) Extraction of uranium(VI) from nitric acid medium by 1.1 M tri-n-butylphosphate in ionic liquid diluent. J Radioanal Nucl Chem 265:31–38
Giridhar P, Venkatesan KA, Subramaniam S, Srinivasan TG, Vasudeva Rao PR (2008) Extraction of uranium (VI) by 1.1 M tri-n-butylphosphate/ionic liquid and the feasibility of recovery by direct electrodeposition from organic phase. J Alloy Compd 448:104–108
Dietz ML, Stepinski DC (2008) Anion concentration-dependent partitioning mechanism in the extraction of uranium into room-temperature ionic liquids. Talanta 75:598–603
Billard I, Ouadi A, Jobin E, Champion J, Gaillard C, Georg S (2011) Understanding the extraction mechanism in ionic liquids: UO2 2+/HNO3/TBP/C4-mimTf2N as a case study. Solvent Extr Ion Exch 29:577–601
Bell TJ, Ikeda Y (2011) The application of novel hydrophobic ionic liquids to the extraction of uranium(VI) from nitric acid medium and a determination of the uranyl complexes formed. Dalton Trans 40:10125–10130
Görller-Walrand C, De Jaegere S (1972) Correlation between the vibronic spectra of the uranyl ion and the geometry of its coordination. Spectroc Acta A 28:257–268
Jørgensen CK, Reisfeld R (1982) Uranyl photophysics. Struct Bond 50:121–171
Denning RG (1992) Electronic structure and bonding in actinyl ions. Struct Bond 79:215–276
Denning RG (2007) Electronic structure and bonding in actinyl ions and their analogs. J Phys Chem A 111:4125–4143
Dai S, Shin YS, Toth LM, Barnes CE (1997) Comparative UV–Vis studies of uranyl chloride complex in two basic ambient-temperature melt systems: the observation of spectral and thermodynamic variations induced via hydrogen bonding. Inorg Chem 36:4900–4902
Hopkins TA, Berg JM, Costa DA, Smith WH, Dewey HJ (2001) Spectroscopy of UO2Cl4 2− in basic aluminum chloride -Ethyl-3-methyl- imidazolium Chloride. Inorg Chem 40:1820–1825
Sornein MO, Cannes C, Le Naour C, Lagarde G, Simoni E, Berthet JC (2006) Uranyl complexation by chloride ions. Formation of a tetrachlorouranium (VI) complex in room temperature ionic liquids [Bmim][Tf2N] and [MeBu3N][Tf2N]. Inorg Chem 45:10419–10421
Servaes K, Hennig C, Billard I, Gaillard C, Binnemans K, Görller-Walrand C, Van Deun R (2007) Speciation of uranyl nitrato complexes in Acetonitrile and in the ionic liquid 1-butyl-3-methylimidazolium Bis (trifluoromethyl- sulfonyl) imide. Eur J Inorg Chem 32:5120–5126
Billard I, Gaillard C, Hennig C (2007) Dissolution of UO2, UO3 and of some lanthanide oxides in BumimTf2N: effect of acid and water and formation of UO2(NO3) −3 . Dalton Trans 37:4214–4221
Georg S, Billard I, Ouadi A, Gaillard C, Petitjean L, Picquet M, Solov’ev V (2010) Determination of successive complexation constants in an ionic liquid: complexation of UO2 2+ with NO3 − in C4-mimTf2N Studied by UV–Vis Spectroscopy. J Phys Chem B 114:4276–4282
Nockemann P, Servaes K, Van Deun R, Van Hecke K, Van Meervelt L, Binnemans K, Görller-Walrand C (2007) Speciation of uranyl complexes in ionic liquids by optical spectroscopy. Inorg Chem 46:11335–11344
Allpress JG, Hambly AN (1959) Infra-Red Spectra of Uranyl Compounds. I. Uranyl Nitrates. Aust J Chem. 12:569–574
Cotton S (1991) Lanthanides and actinides. Oxford University Press, Oxford
Stuart B (2004) Infrared spectroscopy. John Wiley & Sons, Chichester
Woods DA, Bain CD (2014) Total internal reflection spectroscopy for studying soft matter. Soft Matter 10:1071–1096
Quiles F, Burneau A (1998) Infrared and Raman spectroscopic study of uranyl complexes: hydroxide and acetate derivatives in aqueous solution. Vib Spectrosc 18:61–75
Pasilis SP, Pemberton JE (2003) Speciation and coordination chemistry of uranyl(VI)-citrate complexes in aqueous solution. Inorg Chem 42:6793–6800
Lucks C, Rossberg A, Tsushima S, Foerstendorf H, Scheinost AC, Berrnhard G (2012) Aqueous uranium(VI) complexes with acetic and succinic acid: speciation and structure revisited. Inorg Chem 51:12288–12300
Quach DL, Wai CM, Pasilis SP (2010) Characterization of uranyl (VI) nitrate complexes in a room temperature ionic liquid using attenuated total reflection-Fourier transform infrared spectrometry. Inorg Chem 49:8568–8572
Pasilis SP, Blumenfeld A (2011) Effect of nitrate, perchlorate, and water on uranyl (VI) speciation in a room-temperature ionic liquid: a spectroscopic investigation. Inorg Chem 50:8302–8307
Stark A, Behrend P, Braun O, Müller A, Ranke J, Ondruschka B, Jastorff B (2008) Purity specification methods for ionic liquids. Green Chem 10:1152–1161
Liu Y, Chu T, Wang X (2013) A 2:1 dicationic complex of tetraethyl methylenebisphosphonate with uranyl ion in acetonitrile and ionic liquids. Inorg Chem 52:848–854
Görller-Walrand C, De Houwer S, Fluyt L, Binnemans K (2004) Spectroscopic properties of uranyl chloride complexes in non-aqueous solvents. Phys Chem Chem Phys 6:3292–3298
Naito K, Suzuki T (1962) The mechanism of the extraction of several uranyl salts by tri-n-butyl phosphate. J Phys Chem 66:989–995
Gaillard C, El Azzi A, Billard I, Bolvin H, Hennig C (2005) Uranyl complexation in fluorinated acids (HF, HBF4, HPF6, HTf2N): a combined experimental and theoretical study. Inorg Chem 44:852–861
Nakamoto K (2009) Infrared and Raman spectra of inorganic and coordination compounds Part A. Theory and applications in inorganic chemistry. Part A. John Wiley & Sons, Chichester
Nukada K, Naito K, Maeda U (1960) On the mechanism of the extraction of uranyl nitrate by tributyl phosphate II. Infrared Study. Bull Chem Soc Jpn 33:894–898
Freire MG, Carvalho PJ, Gardas RL, Marrucho IM, Santos LM, Coutinho JA (2008) Mutual solubilities of water and the [Cnmim][Tf2N] hydrophobic ionic liquids. J Phys Chem B 112:1604–1610
Noack K, Schulz PS, Paape N, Kiefer J, Wasserscheid P, Leipertz A (2010) The role of the C2 position in interionic interactions of imidazolium based ionic liquids: a vibrational and NMR spectroscopic study. Phys Chem Chem Phys 12:14153–14161
Acknowledgments
We are very grateful to the National Natural Science Foundation of China (Grant No. 91026011 & No. 11575010) for financial support.
Author information
Authors and Affiliations
Corresponding author
Additional information
Yue Wang and Yupeng Liu have contributed equally to this work.
Electronic supplementary material
Below is the link to the electronic supplementary material.
Rights and permissions
About this article
Cite this article
Wang, Y., Liu, Y. & Chu, T. Spectroscopic studies on uranyl complexes with tri-n-butyl phosphate (TBP) in ionic liquids. J Radioanal Nucl Chem 308, 1071–1079 (2016). https://doi.org/10.1007/s10967-015-4608-1
Received:
Published:
Issue Date:
DOI: https://doi.org/10.1007/s10967-015-4608-1