Abstract
Novel interpenetrating polymer networks (IPNs) and semi-interpenetrating polymeric networks (sIPNs) based on polyethyleneimine (PEI) and poly(vinyl alcohol) (PVA) have been prepared via crosslinking reactions with respective crosslinking agent, 1,4-dibromobutane and glutaraldehyde (GA). IPNs, sIPNs and PEI/PVA blend membranes are characterized in detail by Fourier transform Infrared attenuated total reflection (FTIR-ATR) spectroscopy, mechanical properties, water uptake, swelling ratio, field emission scanning electron microscope (FE-SEM), hydroxide ion (OH−) conductivity. Moderate water uptake and swelling ratio are obtained by the IPN derived from PEI:PVA (1:1), achieving 78.4 and 36.8 %, respectively. And the IPN also shows an acceptable OH− conductivity of 4.87 mS/cm at 80 °C.
Similar content being viewed by others
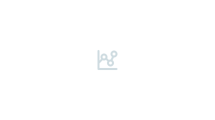
Explore related subjects
Discover the latest articles, news and stories from top researchers in related subjects.Avoid common mistakes on your manuscript.
Introduction
Because of energy depletion and global warming concerns, tremendous attention has been paid to fuel cells, electrochemical energy conversion devices with high conversion efficiency, in the past two decades. Among the various kinds of fuel cells, the anion-exchange membrane fuel cell (AEMFC) is gaining rising interests due to its advantages, such as faster reaction kinetics, usage of non-noble metal catalysts, minimized corrosion problems, lower fuel crossover and more choice of fuel [1]. Hence anion-exchange membranes for fuel cells are being actively studied during the past few years [2]. However, a majority of reports about anion exchange membranes (AEMs) is focused on the development of novel polymer materials with functional groups different from quaternary ammonium [3–21]. Only a few AEM materials were fabricated through modifying commercial polymers [22–25]. Furthermore, to the best of our knowledge, there is not report of IPNs for AEM except for a few works of semi-IPNs.
In this work, we adopted a facile way to prepare AEMs based on PVA and PEI. PVA is rich in hydroxyl groups and has an excellent film-forming property. PEI is abundant in amino groups. Through simple treatment of crosslink, IPNs consisted of PVA and PEI were fabricated. The properties of these novel AEMs were evaluated for AEMFC applications.
Experimental
Materials
PVA (99 + % hydrolyzed, average Mw 89,000 ~ 98,000; Aldrich) and PEI (average Mw 60,000, 50 % solution in H2O; Aldrich) were used as membrane materials. GA, HCl, sodium hydroxide, 1-bromobutane and 1,4-dibromobutane were purchased from Sinopharm Chemical Reagent Co.,Ltd. All reagents from commercial sources were used without further purification.
Preparation of membranes
After 8 wt.% PVA aqueous solution and 8 wt.% PEI aqueous solution were prepared, the two solutions were mixed in the selected PEI/PVA ratios in mass (1:4, 1:3, 1:2, 1:1 and 2:3). The mixture solution was stirred at room temperature till homogeneous cast solution was formed. Then the cast solution was cast on glass plate, and the water was evaporated at 60 °C for 12 h. Thereafter the PEI/PVA blend membrane (transparent and colorless) was peeled off from the substrate, followed by immersion in the mixture of 1,4-dibromobutane and 1-bromobutane for 12 h under ambient conditions. In order to facilitate the crosslink reactions between PEI and 1,4-dibromobutane, the PEI/PVA blend membrane was subsequently put in oven at 120 °C for 12 h. After this treatment, the obtained sIPN (transparent yellow) was soaked in an aqueous solution containing 10 mass% GA and small amount of HCl for 30 min at room temperature to obtain an IPN (red brownish). Finally, the IPN was treated by immersing in 1.0 M NaOH aqueous solution for 24 h, then washed with deionized water until the pH of the effluent water was neutral. The resultant AEMs derived from IPNs were stored in deionized water for measurements.
Measurements
Infrared spectra and membrane morphology measurements
The FTIR-ATR spectroscopy of membrane samples were recorded using a VERTEX70 spectrometer. The surface of the sample was examined through a FE-SEM (Sirion 200). After the sample was dried, it was vacuum-deposited with a thin Pt film for the FE-SEM examination.
Mechanical properties
Tensile strength was measured by using INSTRWN5566 Mechanical Testing Machine. The membrane samples were cut into 0.5 cm × 5.0 cm and examined at an elongation rate of 10 mm /min at room temperature. The tensile strength was calculated with the following equation:
Water uptake and swelling ratio
All membranes were vacuum dried at 120 °C before water uptake and swelling ratio testing. Then the sample films were soaked in deionized water for 24 h at a certain temperature. Weights of dry and wet membranes were measured. The water uptake content was the average of the two measurements with an error within ±3.0 % and calculated by
where ω dry and ω wet are the masses of dried and wet samples, respectively. The swelling ratio was calculated from the length of films by
where l dry and l wet are the lengths of dry and wet samples, respectively.
Hydroxide ion conductivity
Ion conductivity (σ) of the membranes was measured by two-probe AC method from 1Hz to 500 KHz and 10 mV AC perturbation on an Autolab work station. A sample with size of 10 mm × 10 mm was placed in an open, temperature controlled cell where it was clamped between two blocking gilded stainless steel electrodes. Specimens were soaked in deionized water prior to the test. The impedance measurements were performed in water with 100 % relative humidity (RH) at desired temperature. The σ of the membranes in the transverse direction was calculated from the impedance data, using the formula:
where d and A are the thickness and face area of the sample, respectively, and R was the value obtained from the left intersection of the high frequency semi-circle on a complex impedance plane with the Z axis. The conductivities were highly reproducible, and multiple measurements found an average error of <5 %, so this uncertainty is smaller than the plot symbols, hence error bars are omitted.
Results and discussion
Crosslink reaction and FTIR-ATR studies
The schematic diagram of crosslink reactions for forming IPNs and FT-IR spectra of PVA, PEI/PVA blend membrane (PEI:PVA = 1:1), sIPN (PEI:PVA = 1:1) and IPN (PEI:PVA = 1:1) were presented in Figs. 1 and 2. In curve a, the strong and broad absorption peak between 3300 cm−1 and 3500 cm−1 was mainly attributed to the stretching vibration of -OH groups from PVA or the possibly bound water. The peaks located at 1412 and 1086 cm−1 were associated with C-O group. While the peak at 2922 cm−1 could be ascribed to –CH2- group [26, 27]. In curve b, three new bands at 2841 cm−1, 1564 cm−1 and 1092 cm−1 were observed because the PEI was incorporated. They were assigned to –CH2- group, −NH2 group and C-N stretching vibration of PEI, respectively [28–32]. But the characteristic absorbance of the primary and secondary amines of PEI, expected at 3357 cm−1 and 3295 cm−1 for the N-H stretch, were masked by the intense –OH stretching vibration of PVA. In curve c, it was found that the peak at 1564 cm−1 for -NH2 group disappeared, which suggested that the crosslink reaction between PEI and 1,4-dibromobutane occur (Fig. 1a). In curve d, there exhibited the characteristic band at 1140 cm−1, which was corresponding to acetal linkage between the –OH group of PVA and aldehyde group of GA (Fig. 1b) [32–34]. By comparing with curve c, it was obvious that the relative intensity of peaks between 3300 cm−1 and 3500 cm−1 was reduced as a result of the diminution in the number of -OH groups, further confirming the formation of C-O-C bonds.
Water uptake and swelling
Water uptake and swelling play an important role on the ionic conductivity property and dimensional stability of membranes. Higher water uptake leads to higher ionic conductivity, but excess water uptake results in severe damages of mechanical strength. Because the PVA and PEI are soluble in water, the blend membranes begin to dissolve beyond 50 °C and the sIPNs start to dissolve at 80 °C. Hence the water uptake and swelling of samples at 40 °C were plotted in Fig. 3. It could be observed that the water uptake and swelling ratio show similar trends. With the increase of the PVA content, the water uptake and swelling ratio of blend membrane, sIPN and IPN reduced gradually because the higher PVA content led to stronger hydrogen bond interaction. For one PEI/PVA ratio, the water uptake and swelling ratio decreased as follows: blend membrane > sIPN > IPN. But when the temperature was up to 95 °C, only IPNs derived from PEI:PVA (1:1) and PEI:PVA (2:3) could maintain their integrity because the low PEI content might make partial PEI be isolated in PVA and not well interpenetrated with PVA. The swelling ratio of the IPN derived from PEI:PVA (1:1) at different temperatures was shown in Fig. 4. As observed, the swelling ratio increased from 26.3 to 48 % when the temperature increased from 25 to 95 °C.
Mechanical stabilities of the membranes
It is essential for AEMs to maintain the mechanical strength when AEMFC running. The stress–strain curves of the blend membrane, sIPN and IPN derived from PEI:PVA (1:1) were shown in Fig. 5. Because of the flexible structure of PVA and PEI, all the samples had an elongation higher than 300 %. But the maximum stress at break of the blend membrane derived from PEI:PVA (1:1) was no more than 10 MPa. After crosslinking, the maximum stress at break of the sIPN derived from PEI:PVA (1:1) increased to 17.2 MPa and the value of the IPN derived from PEI:PVA (1:1) achieved 24.7 MPa because the crosslinking structure improved the mechanical strength and rigidity of the samples. Furthermore, the maximum stress and elongation at break of the IPN were comparable to those of Nafion 112 [35], indicating that the mechanical properties of the IPN derived from PEI:PVA (1:1) were suitable for the application in AEMFCs.
Morphology
The SEM image of the IPN derived from PEI:PVA (1:1) was shown in Fig. 6. It showed a featureless compact matrix. And no microphase separation was observed even at a magnification of 20000×, suggesting good compatibility between PVA and PEI.
Hydroxide ion conductivity
Ionic conductivity is one of the most important properties of AEMs for AEMFC applications. The OH− conductivity of the IPNs measured at different temperature was shown in Fig. 7. The OH− conductivity exhibited a dependency on temperature similar to proton conduction. The OH− conductivity of all IPNs increased with increasing temperature. This phenomenon could be explained by the fact that elevated temperature was favorable to ion diffusivity, polymeric chain movement and forming broad conduction channels for ion migration. Moreover, according to Fig. 7, it was clear that the OH− conductivity also increased with increasing the content of PEI in IPNs. The IPN derived from PEI:PVA (1:1) displayed the highest OH− conductivity in the whole range of temperature. The best hydroxide ion conductivity achieved was 5.83 mS/cm at 95 °C, which was comparable to the value of a cross-linked quaternized PVA membrane [36].
Conclusions
IPNs comprising PEI and PVA were prepared and compared with the corresponding sIPN and blend membrane. All IPNs demonstrated the lowest water uptake and swelling ration among three kinds of samples. IPN derived from PEI:PVA (1:1) had a water uptake of 78.4 % and a swelling ratio of 36.8 %. It could keep integrity in 95 °C water and possessed mechanical properties comparable to Nafion 112. However, it only exhibited an ionic conductivity of 4.87 mS/cm at 80 °C, so it needs further investigation.
References
Wang Y-J, Qiao J, Baker R, Zhang J (2013) Alkaline polymer electrolyte membranes for fuel cell applications. Chem Soc Rev 42:5768–87
Varcoe JR, Atanassov P, Dekel DR, Herring AM, Hickner MA, Kohl PA et al (2014) Anion-exchange membranes in electrochemical energy systems. Energy Environ Sci 7:3135–91
Fang J, Zhang Y, Wu Y (2012) Preparation and characterization of fluoropolymer anion exchange membrane for alkaline direct methanol fuel cells. Energy Procedia 14:133–6
Xu HK, Fang J, Guo ML, Lu XH, Wei XL, Tu S (2010) Novel anion exchange membrane based on copolymer of methyl methacrylate, vinylbenzyl chloride and ethyl acrylate for alkaline fuel cells. J Membr Sci 354:206–11
Luo Y, Guo J, Wang C, Chu D (2011) Tunable high-molecular-weight anion-exchange membranes for alkaline fuel cells. Macromol Chem Phys 212:2094–102
Zhang Y, Fang J, Wu Y, Xu H, Chi X, Li W et al (2012) Novel fluoropolymer anion exchange membranes for alkaline direct methanol fuel cells. J Colloid Interface Sci 381:59–66
Luo YT, Guo JC, Wang CS, Chu D (2010) Quaternized poly(methyl methacrylate-co-butyl acrylate-co-vinylbenzyl chloride) membrane for alkaline fuel cells. J Power Sources 195:3765–71
Lee H-C, Liu K-L, Tsai L-D, Lai J-Y, Chao C-Y (2014) Anion exchange membranes based on novel quaternized block copolymers for alkaline direct methanol fuel cells. RSC Adv 4:10944–54
Zhang C, Hu J, Wang X, Toyoda H, Nagatsu M, Zhang X et al (2012) Microphase separated hydroxide exchange membrane synthesis by a novel plasma copolymerization approach. J Power Sources 198:112–6
Robertson NJ, Kostalik HA, Clark TJ, Mutolo PF, Abruña HD, Coates GW (2010) Tunable high performance cross-linked alkaline anion exchange membranes for fuel cell applications. J Am Chem Soc 132:3400–4
Liu Z, Zhu X, Wang G, Hou X, Liu D (2013) Novel crosslinked alkaline exchange membranes based on poly(phthalazinone ether ketone) for anion exchange membrane fuel cell applications. J Polym Sci B Polym Phys 51:1632–8
Jheng L-c, Hsu SL-c, Lin B-y, Hsu Y-l (2014) Quaternized polybenzimidazoles with imidazolium cation moieties for anion exchange membrane fuel cells. J Membr Sci 460:160–70
Li C, Wang S, Wang W, Xie X, Lv Y, Deng C (2013) A cross-linked fluorinated poly (aryl ether oxadiazole) s using a thermal cross-linking for anion exchange membranes. Int J Hydrogen Energy 38:11038–44
Wang J, Gu S, Kaspar RB, Zhang B, Yan Y (2013) Stabilizing the imidazolium cation in hydroxide-exchange membranes for fuel cells. ChemSusChem 6:2079–82
Gu F, Dong H, Li Y, Si Z, Yan F (2013) Highly stable N3-Substituted imidazolium-based alkaline anion exchange membranes: experimental studies and theoretical calculations. Macromolecules 47:208–16
Price SC, Williams KS, Beyer FL (2014) Relationships between structure and alkaline stability of imidazolium cations for fuel cell membrane applications. ACS Macro Lett 160–5
Si Z, Qiu L, Dong H, Gu F, Li Y, Yan F (2014) Effects of Substituents and Substitution Positions on Alkaline Stability of Imidazolium Cations and Their Corresponding Anion-Exchange Membranes. ACS Appl Mater Interfaces
Wang W, Wang S, Xie X, Iv Y, Ramani VK (2014) Hydroxide-ion induced degradation pathway for dimethylimidazolium groups in anion exchange membranes. J Membr Sci 462:112–8
Si Z, Sun Z, Gu F, Qiu L, Yan F (2014) Alkaline stable imidazolium-based ionomers containing poly(arylene ether sulfone) side chains for alkaline anion exchange membranes. J Mater Chem A 2:4413–21
Lin X, Liang X, Poynton SD, Varcoe JR, Ong AL, Ran J et al (2013) Novel alkaline anion exchange membranes containing pendant benzimidazolium groups for alkaline fuel cells. J Membr Sci 443:193–200
Li N, Guiver MD, Binder WH (2013) Towards high conductivity in anion-exchange membranes for alkaline fuel cells. ChemSusChem 6:1376–83
Pan J, Li Y, Zhuang L, Lu JT (2010) Self-crosslinked alkaline polymer electrolyte exceptionally stable at 90 degrees C. Chem Commun 46:8597–9
Jasti A, Prakash S, Shahi VK (2013) Stable and hydroxide ion conductive membranes for fuel cell applications: chloromethyaltion and amination of poly(ether ether ketone). J Membr Sci 428:470–9
Qiao J, Fu J, Liu L, Liu Y, Sheng J (2012) Highly stable hydroxyl anion conducting membranes poly(vinyl alcohol)/poly(acrylamide-co-diallyldimethylammonium chloride) (PVA/PAADDA) for alkaline fuel cells: effect of cross-linking. Int J Hydrogen Energy 37:4580–9
Gopi KH, Peera SG, Bhat SD, Sridhar P, Pitchumani S (2014) Preparation and characterization of quaternary ammonium functionalized poly(2,6-dimethyl-1,4-phenylene oxide) as anion exchange membrane for alkaline polymer electrolyte fuel cells. Int J Hydrogen Energy 39:2659–68
Wang ED, Zhao TS, Yang WW (2010) Poly (vinyl alcohol)/3-(trimethylammonium) propyl-functionalized silica hybrid membranes for alkaline direct ethanol fuel cells. Int J Hydrogen Energy 35:2183–9
Wu S, Li F, Wang H, Fu L, Zhang B, Li G (2010) Effects of poly (vinyl alcohol) (PVA) content on preparation of novel thiol-functionalized mesoporous PVA/SiO2 composite nanofiber membranes and their application for adsorption of heavy metal ions from aqueous solution. Polymer 51:6203–11
Moradian H, Fasehee H, Keshvari H, Faghihi S (2014) Poly(ethyleneimine) functionalized carbon nanotubes as efficient nano-vector for transfecting mesenchymal stem cells. Colloids Surf B 122:115–25
Park SH, Yang HN, Lee DC, Park KW, Kim WJ (2014) Electrochemical properties of polyethyleneimine-functionalized Pt-PEI/carbon black as a catalyst for polymer electrolyte membrane fuel cell. Electrochim Acta 125:141–8
Wen S, Zheng F, Shen M, Shi X (2013) Surface modification and PEGylation of branched polyethyleneimine for improved biocompatibility. J Appl Polym Sci 128:3807–13
Leroy D, Martinot L, Mignonsin P, Strivay D, Weber G, Jérôme C et al (2003) Complexation of uranyl ions by polypyrrole doped by sulfonated and phosphonated polyethyleneimine. J Appl Polym Sci 88:352–9
Srinivasa Rao P, Smitha B, Sridhar S, Krishnaiah A (2006) Preparation and performance of poly(vinyl alcohol)/polyethyleneimine blend membranes for the dehydration of 1,4-dioxane by pervaporation: comparison with glutaraldehyde cross-linked membranes. Sep Purif Technol 48:244–54
Devi DA, Smitha B, Sridhar S, Jawalkar SS, Aminabhavi TM (2007) Novel sodium alginate/polyethyleneimine polyion complex membranes for pervaporation dehydration at the azeotropic composition of various alcohols. J Chem Technol Biotechnol 82:993–1003
Tsai CE, Lin CW, Hwang BJ (2010) A novel crosslinking strategy for preparing poly(vinyl alcohol)-based proton-conducting membranes with high sulfonation. J Power Sources 195:2166–73
Miyatake K, Zhou H, Watanabe M (2004) Proton conductive polyimide electrolytes containing fluorenyl groups: synthesis, properties, and branching effect. Macromolecules 37:4956–60
Xiong Y, Fang J, Zeng QH, Liu QL (2008) Preparation and characterization of cross-linked quaternized poly(vinyl alcohol) membranes for anion exchange membrane fuel cells. J Membr Sci 311:319–25
Author information
Authors and Affiliations
Corresponding author
Rights and permissions
About this article
Cite this article
Zuo, D., Gong, Y., Yan, Q. et al. Preparation and characterization of hydroxyl ion-conducting interpenetrating polymer network based on PVA and PEI. J Polym Res 23, 126 (2016). https://doi.org/10.1007/s10965-016-1020-7
Received:
Accepted:
Published:
DOI: https://doi.org/10.1007/s10965-016-1020-7