Abstract
Poly (glycerol sebacate) (PGS) is a synthetic polymeric material with the characteristics of controllable degradation, high plasticity and excellent biocompatibility. However, the time of PGS degradation is faster than that of cartilage regeneration, which limits its application in cartilage tissue engineering. Polycaprolactone (PCL), a widely used synthetic polymer, has appropriate biodegradability and higher mechanical strength. This study aims to make a scaffold from blends of fast degrading PGS and slowly degrading PCL, and to investigate its potential for cartilage tissue engineering applications. Scanning electron microscopic analysis indicated that the scaffolds provided favourable porous microstructures. In vitro degradation test showed that PGS/ PCL scaffolds acquired longer degradation time and better mechanical strength. PGS/PCL scaffolds seeded with Bone marrow-derived mesenchymal stem cells (BMSCs) and articular chondrocytes (ACCs) were cultured in vitro. Short-term in vitro experiments confirmed that both seeded cells could adhere and proliferate on the scaffold. Chondrogenic culture for cell-scaffold constructs confirmed BMSCs could differentiate into chondrocyte-like cells in PGS/PCL scaffolds. With tunable biodegradation, favorable mechanical properties and cytocompatibility, PGS/PCL scaffolds would potentially be suitable for the regeneration of cartilage tissue.

Poly (glycerol sebacate) (PGS) is a synthetic polymeric material with the characteristics of controllable degradation, high plasticity and good biocompatibility. However, the time of PGS degradation is faster than that of cartilage regeneration, which limits its application in cartilage tissue engineering. Polycaprolactone(PCL), a widely used synthetic polymer, has appropriate biodegradability. This study aims to make a scaffold from blends of fast degrading PGS and slowly degrading PCL, and to investigate its potential for cartilage tissue engineering applications. Scanning electron microscopic analysis indicated that the scaffolds provided favourable porous microstructures. In vitro degradation test showed that PGS/ PCL scaffolds got longer degradation time with surface degradation nature. PGS/PCL scaffolds seeded with Bone marrow-derived mesenchymal stem cells (BMSCs) and articular chondrocytes (ACCs) were cultured in vitro under the same condition. Short-term in vitro experiments confirmed that both seed cells could adhere and proliferate on the scaffold. Chondrogenic culture for cell-scaffold constructs confirmed BMSCs could differentiate into chondrocyte-like cells and form cartilage-specific matrix in PGS/PCL scaffolds. With cytocompatibility and biodegradation profile, PGS/PCL scaffolds get great potential for cartilage tissue engineering.
Similar content being viewed by others
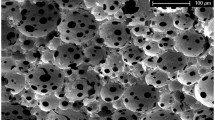
Explore related subjects
Discover the latest articles, news and stories from top researchers in related subjects.Avoid common mistakes on your manuscript.
1 Introduction
Articular cartilage injury is a common clinical disease, if not handled properly will lead to joint noose, joint dysfunction, and ultimately osteoarthritis [1]. If the injury do not acquire effective treatment, it may lead to joint noose, joint dysfunction, and ultimately osteoarthritis. In general, the superficial cartilage injury will not recover by itself, while the full-layer cartilage injury will lead to fibrocartilage healing, which is inferior to native hyaline cartilage [2]. At present, common clinical treatment methods include joint cavity cleaning, microfracture, autogenous bone cartilage transplantation (such as mosaic transplantation), and allograft bone cartilage transplantation. But none of them has yet proved to be quite successful, as they all have drawbacks and limitations. Microfracture can not provide sufficient tissue regeneration and recovery to original functions. Cartilage transplantation is limited by immunological rejection, donor site morbidity and insufficient donor supply [3,4,5]. Other methods have shown promise but are far from perfect. Therefore, it is of great clinical significance to study a method that can effectively promote the repair of cartilage injury.
With the development of material science and life science, the application of bio-absorbable materials to repair cartilage injury has become a hot topic in the field of tissue engineering. Tissue engineering is based on the high concentration of cells inoculated on biomaterials to make cell-scaffold constructs. The cell-scaffold construct is then implanted into the injury site to repair or replace the damaged tissue [6]. Tissue engineering scaffold, as a carrier of cells, plays an important role in tissue regeneration [7].
According to the study of Wang et al. in 2002, poly (glycerol sebacate) (PGS) is a kind of polymeric material, which is synthesized by condensation reaction of glycerol and sebacic acid at 1:1 molar ratio [8]. PGS, as tunable biodegradable elastomeric polyester, is widely used in myocardial, blood vessels, nerves, drug delivery and other medical research. Biocompatibility studies of PGS have shown improved cell attachment, spreading and proliferation [9,10,11,12,13,14]. The surface degradation nature of PGS makes it preferable for scaffolding application so as to maintain the integrity of scaffolds in the process of degradation [15]. Moreover, glycerol and sebacic acid, as synthetic substrates and degradation products of PGS, are also bioresorbable. Comparing to other synthetic polymers such as poly(lactic acid)(PLA) and poly(lactic-co-glycolic acid) (PLGA), PGS triggered lower inflammatory responses and minimal fibrous encapsulation [16,17,18]. PGS also has high plasticity. The mechanical properties and degradation ability can be changed by adjusting the synthesis conditions and reactant concentration. However, the time of PGS degradation is faster than that of cartilage regeneration, which limits its application in cartilage tissue engineering [9]. Poly(caprolactone) (PCL), as a widely used slowly degrading synthetic polymer, has appropriate biodegradability and mechanically stiffness [19,20,21,22], which makes up for the shortage of PGS. However, it shows poor cell behavior due to its hydrophobicity.
In this study, we presented a salting-out method for the generation of PGS/PCL blends scaffolds. We further compared mechanical properties and biodegradability of PGS/PCL scaffolds with those made from PGS or PCL. Bone marrow mesenchymal stem cells (BMSCs) and articular chondrocytes (ACCs),as seeded cells, have been used in a variety of cartilage tissue engineering scaffolds【参考文献】. The rabbit BMSCs and ACCs were seeded on PGS/PCL scaffolds respectively and the biocompatibility and chondrogenic potential of the scaffolds were accessed.
2 Materials and methods
2.1 Synthesis of PGS
PGS were synthesized according to the method previously reported by Wang et al. [8]. It can be divided into two stages: pre-polymerization reaction and curing drying. First, glycerol (Sigma-Aldrich, USA) and sebacic acid (Sigma-Aldrich, USA) were mixed in equal molar ratio and then added to the reactor (four-neck bottle). The mixture was heated at 135 °C for 24 h under nitrogen protection. The reactor was then vacuum pumped and heated for 48 h at 135 °C for 48 h. After cooling, the pre-polymer was obtained (Fig. 1).
2.2 Preparation of PGS/PCL scaffolds
PGS/PCL porous scaffolds were prepared by salting out method. First, sodium chloride was crushed and grinded by a grinder and the screen mesh was used to screen out sodium chloride particles with 150–200 um diameter. The particles were placed in a circular metal mold to make a salt mold. Second, PGS prepolymer was mixed with PCL (Mn = 70,000–90,000 g/mol) (Daigang, China) in a weight ratio of 1:1 and the mixture was dissolved in tetrahydrofuran (THF) solution (100 mg/mL). The PGS/PCL blend solutions were placed in the salt mold and put into 150 °C vacuum oven to crosslink 24 h.Then the PGS/PCL mixture was immersed in distilled water to ensure that the sodium chloride particles are fully dissolved. Finally, PGS/PCL porous tissue engineering scaffolds were obtained by freeze-drying for 48 h. The porosity (P) of the scaffolds was calculated according to the formula: Vp/Vs × 100 (Vp: the pore volume, Vs: the scaffold volume). Vp was calculate on Archimedes principle.
2.3 Surface morphology of porous scaffolds
The surface morphology of the scaffold was observed by a QUANTA 450 scanning electron microscope (SEM). The samples were placed on the 8 mm diameter aluminum platform and sputtered with gold-palladium. Three samples were observed and analyzed.
2.4 In vitro degradation experiments
The PGS/PCL porous scaffolds (10 mm in diameter and 4 mm in thickness) were soaked in the phosphate buffer solution (PBS) and the PBS was replaced every 24 h. Formula for calculating mass loss: η = ((Wo − Wt)/Wo) × 100%.
Wo represented the initial weight of the scaffolds. Wt represented their weight after soaking time. Three scaffolds were collected from each time point.
2.5 Mechanical properties
For mechanical characterization of the scaffolds, PGS, PCL, and PGS-PCL scaffolds with the same porous structure were studied by subjecting the degraded scaffolds to uniaxial mechanical testing. All the scaffolds were cut into rectangular shapes (15 × 5 mm2) and soaked in the PBS for degradation test. After each time point (0, 1, 2, 3, 5, 7 days),degraded scaffolds were collected for uniaxial mechanical test with universal tester (YIHUAN YHS-216, China). The samples were loaded at a rate of 7 mm/min and the elastic modulus (EM) was measured using the Young’s modulus based on the linear portion of the stress–strain curve.
2.6 Isolation and culture of BMSCs and ACCs
All animals are provided by the animal laboratory center of Dalian Medical University. All animal experimental protocols were approved by the ethics committee of Dalian Medical University.
The bone marrow blood was extracted from the femur and tibia of New Zealand rabbits weighing 1.5–2 kg, and diluted with complete growth medium (DMEM/F12, 10% FBS, 1% penicillin and streptomycin) to avoid blood clotting. Bone marrow suspension was inoculated in cell culture bottles and incubated in complete growth medium at 37 °C, 5% CO2. The complete growth medium was replaced every three days.
The cartilage samples were extracted from the knee and shoulder joint of the rabbits and digested for 12 h with 2 mg/ml collagenase type 2 solutions (Solarbio, USA) at 37 °C. The obtained cells were cultured in complete growth medium (DMEM/F12, 10% fetal bovine serum, 1% penicillin and streptomycin) at 37 °C, 5% CO2. The complete culture medium was replaced every three days. Chondrocytes were used for subsequent experiments after passages.
2.7 Cell seeding and cell-scaffold constructs culture
The PGS/PCL scaffolds were seeded with BMSCs or ACCs respectively as Fig. 1. All scaffolds were cut into disks (5 mm in diameter and 1 mm in thickness) for the culture of BMSCs and ACCs. All scaffolds were pretreated with 75% ethanol solution and disinfected for 24 h. Then washed repeatedly by sterile phosphate buffered saline (PBS) to ensure that residual ethanol was removed. The third generation of BMSCs and ACCs were fused to 80% and then trypsinized with 0.05% trypsin solution (Solarbio, USA) respectively. Forty microliter cell suspension at a concentration of 5 × 105 cell/scaffold was implanted on the scaffolds for 4 h. Then appropriate amount of the complete growth medium was added. The cell-scaffold constructs were cultured at 37 °C in a humidified atmosphere containing 5% CO2 and culture medium was changed every 3 days until the cell-scaffold constructs were sent for subsequent analyses.
2.8 Cell proliferation ability test
The proliferation of BMSCs and ACCs on PGS/PCL scaffolds was detected by CCK-8 assay (Dojindo, Japan). After 1, 3, 5, and 7 days of cell-scaffold constructs culture, the cells were transferred to the 96-well plate and incubated with 100 µl medium and 10 µl CCK-8 solution for 3 h. The absorbance of supernatant in 450 nm was measured by microplate reader (Multiskan Spectrum, Thermo Fisher, USA) to evaluate cell proliferation. Three separate experiments were performed for each time point.
2.9 Cell viability testing
The viability of BMSCs and ACCs in the scaffolds was evaluated by a LIVE/DEAD Viability/Cytotoxicity Kit assay (Invitrogen, Carlsbad, CA, USA). The cell-scaffold constructs were cultured in complete culture medium for 3 and 7 days, respectively. Using sterile PBS to wash cell-scaffold constructs. The cell-scaffold constructs were incubated in proper amount of working liquid to ensure that all constructs were immersed in the solution for 1 h. The cell-scaffold constructs were observed under laser confocal microscope (LEICA TCS SP 8) at 568 and 488 nm, respectively. The cell-free scaffolds were used as the control group to avoid the background effect of the scaffolds.
2.10 Cell morphology detection
Cell-scaffold constructs were cultured in vitro for 7 days. The constructs were washed with PBS and fixed with 4% paraformaldehyde for 30 min. And 0.1% Triton X-100 solution was used to permeate the cell membrane. The cytoskeleton was stained by rhodamine phalloidin (KeyGEN, China) for 30 min at 37 °C and the nuclei were stained with Hoechst33258 working solution (Sigma-Aldrich, USA) for 10 min. Cytoskeleton protein was observed by confocal microscope.
2.11 Cell distribution
The adhesion of BMSCs and ACCs in the scaffolds was observed by SEM. Cell-scaffold constructs were cultured in vitro for 24 h and non-seeded scaffolds were used as control group. All scaffolds were fixed with 2.5% glutaraldehyde for 12 h and then dehydrated with gradient alcohol. The scaffolds were vacuum-coated with gold in a high-vacuum gold spatter coater and observe with electron microscope.
2.12 Chondrogenic culture of cell-scaffold constructs
BMSCs and ACCs were inoculated in the PGS/PCL scaffolds (10 mm in diameter and 1 mm in thickness), respectively. Each scaffold was implanted with 50 µl cell suspension with 5 × 105 cells. Both the BMSCs- and the ACCs-scaffolds were fed with chondrogenic differentiation medium (Basal Medium 200 ml, Dexamethasone 20 µl, Ascorbate 600 µl, ITS + Supplement 2 ml, Proline 200 µl, TGF-β3 2 ml) (cyagen, USA) after 3 days of culture in complete growth medium. The medium is changed every 2–3 days until the cell-scaffold constructs were sent for subsequent analyses.
2.13 Histological analysis
In vitro chondrogenic culture for 21 days, cell-scaffold constructs were harvested and fixed in 4% paraformaldehyde (PFA) for 1 h and then dehydrated by 30% sucrose solution. After snap freezing in optimal cutting temperature (O.C.T), sections were washed in distilled water to remove O.C.T and then stained for glycosaminoglycans with toluidine blue for 15 min before washing in distilled water.
2.14 Biochemical analysis for chondrogenic culture cells
The DNA content of cells in the scaffolds was quantified using Hochest33258 fluorescence staining assay (Sigma-Aldrich, USA) following the manufacturer’s instructions. In vitro Chondrogenic culture for 7, 14, and 21 days, cell-scaffold constructs (10 mm in diameter and 1 mm in thickness) were digested in 250 µg/ml papain solution (Merck, USA) for 48 h. The 40 µl supernatant was mixed with 160 µl Hochest33258 working solution (2 µg/ml) for 20 min in darkroom. The fluorescence intensity of the solution was measured by spectrofluorometer (Infinite M200 Pro, Tecan, Switzerland). The content of DNA was calculated according to the standard curve of DNA concentration.
1, 9-dimethylmethylene blue dye-binding assay (PH 1.75, Sigma Aldrich, USA) (DMMB) was used to quantify glycosaminoglycan (GAG) content secreted by the cells in the scaffolds. The supernatant was added to the 96-well plate with 200 µl DMMB working solution. The absorbance of the samples was determined by a microplate reader (Multisk-an Spectrum, Thermo Fisher, USA) at 525 nm. The GAG content was measured using a standard curve from chondroitin sulfate C (Solarbio, China). The content of GAG was normalized according to the content of DNA.
2.15 Gene expression analyses for chondrogenic culture cells
Cell-scaffold constructs were collected on 7, 14, and 21days. Total RNA was extracted by RNAiso Plus (Takara, Japan). PrimeScript™ RT reagent Kit (Takara, Japan) was used to reverse the extracted RNA to cDNA. SYBR Premix EX Taq II Kit (Takara, Japan) was used to carry out RT-PCR on a Stepone plus real-time PCR machine (Applied Biosystems, USA). The reaction conditions are: predenaturation at 95 °C for 5 min and then a cycle of 40 at 95 °C for 30 s and 55 °C for 30 s. The expression of related chondrogenic genes in the samples was normalized by the housekeeping gene glyceraldehyde-3-phosphate dehydrogenase (GAPDH). PCR primers are listed.
2.16 Statistical analysis
All quantitative data were presented as the means ± standard deviation and analyzed by analysis of variance (ANOVA) and post hoc Tukey’s tests. P-values < 0.05 were considered statistically significant. Means and standard deviations are shown on each figure. Statistical analysis was performed using SPSS-20.
3 Result
3.1 Synthesis of PGS and preparation of PGS/PCL porous scaffolds
Yellowish viscous PGS prepolymer was generated by the polycondensation of glycerol and sebacic acid. PGS prepolymer was mixed with PCL in THF and heated to 150 °C for 24 h. The porous scaffolds were prepared by salting-out method. Figure 1 shows the SEM images of porous scaffolds. It can be seen that the pore size was between 100–250 µm and the average porosity was 85.4 ± 7.5% (n = 10).
3.2 Scaffolds degradation and mechanical properties
Figure 2a showed the result of the mass loss of PGS/PCL scaffolds in PBS. Statistical analysis revealed significant differences between the three scaffolds of weight loss for all time points. The weight loss rate of PGS/PCL scaffolds were significantly slower than that of pure PGS stent, and a little faster than that of PCL. In the early stage of degradation, the weight loss of PGS/PCL scaffolds were fast and the weight loss in 10 days was 8 + 2.43%. After 10 days, the degradation rate decreased and the curve tended to be slow.
Physical characterization of the PGS/PCL scaffolds compared to the PGS and PCL scaffold. a Degradation characterization of the scaffolds. PGS/PCL scaffolds showed more stability under hydrolytic degradation (n = 6, p < 0.05). b–d Tensile mechanical properties of the sacffolds during the degradation (n = 6)
Mechanical properties of PGS, PCL and PGS-PCL scaffolds during degradation in vitro are shown in Fig. 2. Obviously, the elastic modulus (EM) of PGS/PCL scaffolds during degradation was higher than PGS group (p < 0.05). And EM value also showed significant change from 3.13 ± 0.25 MPa to 2.228 ± 0.13 MPa after 7 days degradation while those PGS scaffolds also showed significant decrease in the mechanical properties after 7 days. On the other hand, PCL scaffolds showed the highest EM value in the three group and there was no significant changes in the EM value after 7 days during degradation (p > 0.05).
3.3 Cell attachment, distribution, proliferation, and viability
The cell-scaffold constructs were cultured in complete growth medium in vitro.CCK-8 assay was used to detect the proliferation of cells in the scaffolds (Fig. 4). Compared with the first day, the number of BMSCs and ACCs in the scaffolds increased significantly. After cultured in vitro for 7 days, no significant difference was found among two groups.
The results of electron microscope (SEM) showed that BMSCs and ACCs were attached to the PGS/PCL scaffolds along the edge of the micropores after 24 h.
BMSCs showed polygonal or fusiform morphology and ACCs showed polygonal and cobblestone-like morphology (Fig. 3).
The results of cytoskeleton staining in vitro for 7 days showed that the BMSCs maintained the typical fusiform morphology and the chondrocytes showed polygonal (Fig. 4a, b).
The cell viability was detected at 3 days and 7 days, respectively (Fig. 5). After 3 days culture, BMSCs and ACCs were homogeneously distributed in scaffolds and most of them were living cells. After 7 days of culture in vitro, the number of cells in both groups was significantly higher than that in the third day and a small number of dead cells appeared in the place with high cell density. There was no significant difference in the proportion of living cells between ACCs and BMSCs (n = 5, P > 0.05, Fig. 5 living cell/dead cell column).
3.4 Cartilage induced culture results
In order to qualitatively evaluate the progressive chondrogenic differentiation of BMSCs, histology was performed on cell/scaffold constructs (Fig. 6). Toluidine blue of glycosaminoglycans showed positivity in ACCs and weakly positivity in BMSCs. No apparent extracellular matrix deposition was found in the sections (Fig. 6a, b).
After 21 days in vitro culture, the DNA content of both cell-scaffold constructs, measured through a Hoechst dye 33258 methods, were increased gradually (Fig. 7a). However, there were no significant differences in DNA content between BMSCs and ACCs in vitro culture. This showed a similar trend to the proliferation result of CCK8 assay.
Cartilage induced culture results. a Amount of DNAs per construct during 21 days for BMSCs-scaffold constructs and ACCs-scaffold constructs. b Glycosaminoglycan (GAG) content per construct was assessed by DMMB assay. c The content of GAG was standardized according to the content of DNA, (n = 5, *p < 0.05)
Glycosaminoglycan (GAG) content was used to quantify cartilaginous matrix production by cells cultured with chondrogenic differentiation medium for 21 days (Fig. 7b). And GAG content was standardized by the content of DNA in the scaffold at different time points (Fig. 7c). The GAG content in ACCs group was significantly higher than that in BMSCs group at 7 and 14 days. However, the GAG content in ACCs group decreased after 7 days. And the GAG content in BMSCs groups increased continuously in the 21 days. Finally the GAG content in BMSCs groups reached a higher level than the ACCs group at day 21.
Cartilage specific genes (COL2, AGC, SOX9) and chondrocyte dedifferentiation marker genes (COL1) were detected by quantitative polymerase chain reaction (Q-PCR) The gene expression of the cell-scaffold constructs was significantly different at different time points (Fig. 8). In the BMSCs group, COL2 AGC and COL1 expression increased gradually and SOX9 expression decreased gradually during the 21 days chondrogenic culture. In the ACCs group, cartilage specific genes (COL2, AGC, SOX9) and chondrocyte dedifferentiation marker genes (COL1) expression were at a high level. However, the above gene expression decreased gradually during the 21 days chondrogenic culture. In addition, COL2 gene expression of BMSCs group was higher than that of ACCs group at 21 days. The AGC SOX9 and COL1 expression of BMSCs and ACCs groups were not significantly different from each other at 21 days.
4 Discussion
Seed cells, scaffold materials and growth factors are the essential components of tissue engineering. Tissue engineering scaffold, the carrier of seed cells, plays an important role in the process of damage repair. The ideal cartilage tissue engineering scaffold should have the following characteristics [23,24,25,26]: (1) Good histocompatibility; (2) Biodegradability and the degradation products should be absorbed by human body, without causing inflammatory reaction and immune reaction; (3) Porous structure with high porosity and suitable pore size; (4) Easy synthesis and processing; (5) Mechanical strength and elasticity.
Poly (glycerol sebacate) (PGS), as a kind of synthetic polymeric material, has characterizes of good mechanical properties、high plasticity and easy processing. Besides, PGS has biodegradability which was reported to be degraded via a surface erosion mechanism [9] and great biocompatibility which has been used as tissue engineering scaffolds for many other fields [27, 28]. In addition, PGS contains a large number of hydroxyl and carboxyl groups, giving the PGS better biological characteristics. Polycaprolactone (PCL) is a classic polymeric material with tough mechanical properties and longer degradation time which just makes up for the deficiencies of PGS.
In this study, PGS/PCL porous scaffolds were made with PGS and PCL by salting-out method. The results showed that PGS/PCL mixed material had great potential as a scaffold for fibrocartilage regeneration. According to a study, porous structure is essential for nutrient/gas transport and consecutively for cell response [29]. As shown in our study, PGS/PCL material could be fabricated into 3D-designed porous scaffolds with the porosity of 85.4 + 7.5%. According to SEM images, the scaffolds exhibited porous structure and the diameter of the pores were 100–250 μm which were the same size of sodium chloride particles in the salt mold.
The degradation test data revealed degradation rate of PGS/PCL scaffolds was significantly slower than that of PGS scaffolds which was slow enough to allow for the regeneration of extracellular matrix (ECM) and restoration of mechanical integrity. Additionally, PGS/PCL scaffolds could maintain the integrity of structure during the degradation process. The degradation mode of PGS/PCL scaffold was considered to be surface degradation mode. Thus, the combination of these two polymers fulfils the initial physical requirements to be used as tissue engineering scaffolds in cartilage regeneration.
BMSCs and ACCs are commonly used as seeded cells in cartilage tissue engineering [30]. In vitro experiment showed that PGS/PCL scaffolds could indeed be used for cartilage tissue engineering applications where BMSCs and ACCs were seeded in. According to SEM observation, two groups of cells readily attached and spread on PGS/PCL scaffolds surface and inner pores. The result of CCK-8 assay indicated that the number of BMSCs and ACCs in the scaffolds gradually increased in vitro culture for 7 days. The high vitality percentage and quite low apoptosis and necrosis rates observed in both group indicate that BMSCs and ACCs displayed excellent cell viability, adhesion ability and proliferation potential in the scaffolds. There is no significantly different in adhesion, viability and proliferation between BMSCs and ACCs. The PGS/PCL scaffolds exhibited great cytocompatibility with BMSCs and ACCs in vitro culture for short term. This is in agreement with previous studies of PGS-PCL scaffolds seeded with other cells, such as C2C12 mouse myoblasts, primary human valvular interstitial and human corneal endothelial cells [14].
Cartilage induced culture results from expression of cartilage-specific genes and GAG quantitative detection showed the differentiation of BMSCs into chondrocytes. Healthy articular cartilage is composed of a highly organized network of collagen and proteoglycans. Type II collagen is the major collagen of articular cartilage and aggrecan is the main proteoglycan in cartilage [31]. COL2 and AGC are considered to be typical markers of differentiated chondrocytes. Besides, SOX9 is thought to function as a transcriptional regulatory factor that plays an important role in proliferation, differentiation and extracellular matrix secretion of chondrocytes. In the early stage of cartilage induction, chondrogenic genes (COL2, AGC, and SOX9) expression of ACCs group was significantly higher than that of BMSCs. After being cultured in chondrogenic medium for 4 weeks, COL2 and AGC expression increased gradually in BMSCs group and decreased in ACCs group. Finally COL2 expression of BMSCs group was higher than that of ACCs group at 21 days. The same trend was found in the AGC gene expression level between the BMSCs and ACCs groups. In addition to the cartilage-specific gene expression, the GAG content of BMSCs group also increased during 21 days. These results indicated BMSCs could differentiate into chondrocyte-like cells and form cartilage-specific matrix in PGS/PCL scaffolds. Cartilage-specific gene expression level and GAG content of BMSCs-scaffold group increased with time but they are still less than that of early passage (7 days) of ACCs-scaffolds.
In contrast to the BMSCs, we found that the ACCs seeded in the scaffolds were losing chondrocyte phenotypes, which may explain why the GAG content and chondrogenic genes (COL2, AGC, SOX9) expression level decreased during the 21 days. Notably,the SOX9 genes expression of BMSCs group also slightly decreased, which indicated that differentiated BMSCs might have a tendency to differentiate towards a hypertrophic phenotype. The COL1 genes expression level of both groups were relatively lower than chondrogenic genes (COL2, ACG).That means most of BMSCs and ACCs seeded in PGS/PCL scaffolds still maintained chondrocyte phenotypes.
The in vitro results showed PGS/PCL scaffold had a significant potentiality for cartilage tissue engineering. However, these results must be verified by in vivo studies, since microenvironment in vivo may affect chondrogenic ability. Future work will address this study’s limitations.
5 Conclusion
PGS is a biocompatible and biodegradable elastomeric polymer that has emerged as a promising scaffold material for tissue engineering applications. However too fast degradation and low stiffness limit its application in cartilage tissue engineering. In our study, PGS/PCL composite porous scaffolds were successfully fabricated. While maintaining biocompatible and biodegradable of PGS, PCL was used to prolong the degradation time and increase stiffness. Composite scaffolds possess better mechanical properties and longer degradation time compared to PGS scaffolds. In addition, PGS/PCL scaffolds exhibited outstanding cytocompatibility with both BMSCs and ACCs in vitro. BMSCs successfully underwent chondrogenesis in the PGS/PCL scaffolds with chondrogenic differentiation medium and differentiated BMSCs got similar chondrogenic ability as ACCs. The physical and biological advantages of this composite scaffold can motivate further studies for potential applications in cartilage tissue engineering.
References
Ge Z, Hu Y, Heng BC, Yang Z, Ouyang H, Lee EH. et al. Osteoarthritis and therapy. Arthritis Rheum. 2006;55:493–500.
Jorgensen C, Gordeladze J, Noel D. Tissue engineering through autologous mesenchymal stem cells. Curr Opin Biotechnol. 2004;15:406–10.
Acharya C, Adesida A, Zajac P, Mumme M, Riesle J, Martin I. et al. Enhanced chondrocyte proliferation and mesenchymal stromal cells chondrogenesis in coculture pellets mediate improved cartilage formation. J Cell Physiol. 2012;227:88–97.
Darling EM, Athanasiou KA. Retaining zonal chondrocyte phenotype by means of novel growth environments. Tissue Eng. 2005;11:395–403.
Levorson EJ, Levorson EJ, Hu O, Mountziaris PM, Kasper FK, Mikos AG. Cell-derived polymer/extracellular matrix composite scaffolds for cartilage regeneration, Part 2: construct devitalization and determination of chondroinductive capacity. Tissue Eng Part C Methods. 2014;20:358–72.
Yang S, Leong KF, Du Z, Chua CK. The design of scaffolds for use in tissue engineering. Part II. Rapid prototyping techniques. Tissue Eng. 2002;8:1–11.
Yoo HS, Lee EA, Yoon JJ, Park TG. Hyaluronic acid modified biodegradable scaffolds for cartilage tissue engineering. Biomaterials. 2005;26:1925–33.
Wang Y, Ameer GA, Sheppard BJ, Langer R. A tough biodegradable elastomer. Nat Biotechnol. 2002;20:602–6.
Wang Y, Kim YM, Langer R. In vivo degradation characteristics of poly(glycerol sebacate). J Biomed Mater Res A. 2003;66:192–7.
Rai R, Tallawi M, Barbani N, Frati C, Madeddu D, Cavalli S. et al. Biomimetic poly(glycerol sebacate) (PGS) membranes for cardiac patch application. Mater Sci Eng C Mater Biol Appl. 2013;33:3677–87.
Hu J, Kai D, Ye H, Tian L, Ding X, Ramakrishna S. et al. Electrospinning of poly(glycerol sebacate)-based nanofibers for nerve tissue engineering. Mater Sci Eng C Mater Biol Appl. 2017;70(Pt 2):1089–94.
Salehi S, Czugala M, Stafiej P, Fathi M, Bahners T, Gutmann JS. et al. Poly (glycerol sebacate)-poly (epsilon-caprolactone) blend nanofibrous scaffold as intrinsic bio- and immunocompatible system for corneal repair. Acta Biomater. 2017;50:370–80.
Wang M, Lei D, Liu Z, Chen S, Sun L, Lv Z. et al. A poly(glycerol sebacate) based photo/thermo dual curable biodegradable and biocompatible polymer for biomedical applications. J Biomater Sci Polym Ed. 2017;28:1728–39.
Rai R, Tallawi M, Frati C, Falco A, Gervasi A, Quaini F. et al. Bioactive electrospun fibers of poly(glycerol sebacate) and poly(epsilon-caprolactone) for cardiac patch application. Adv Healthc Mater. 2015;4:2012–25.
Kerativitayanan P, Gaharwar AK. Elastomeric and mechanically stiff nanocomposites from poly(glycerol sebacate) and bioactive nanosilicates. Acta Biomater. 2015;26:34–44.
Motlagh D, Yang J, Lui KY, Webb AR, Ameer GA. Hemocompatibility evaluation of poly(glycerol-sebacate) in vitro for vascular tissue engineering. Biomaterials. 2006;27:4315–24.
Gaharwar AK, Nikkhah M, Sant S, Khademhosseini A. Anisotropic poly (glycerol sebacate)-poly (-caprolactone) electrospun fibers promote endothelial cell guidance. Biofabrication. 2014;7:015001.
Sant S, Iyer D, Gaharwar AK, Patel A, Khademhosseini A. Effect of biodegradation and de novo matrix synthesis on the mechanical properties of valvular interstitial cell-seeded polyglycerol sebacate-polycaprolactone scaffolds. Acta Biomater. 2013;9:5963–73.
Sodian R, Hoerstrup SP, Sperling JS, Daebritz S, Martin DP, Moran AM. et al. Early in vivo experience with tissue-engineered trileaflet heart valves. Circulation. 2000;102(19 Suppl 3):III22–9.
Del Gaudio C, Bianco A, Grigioni M. Electrospun bioresorbable trileaflet heart valve prosthesis for tissue engineering: in vitro functional assessment of a pulmonary cardiac valve design. Ann Ist Super Sanita. 2008;44:178–86.
Bragta P, Sidhu RK, Jyoti K, Baldi A, Jain UK, Chandra R. et al. Intratumoral administration of carboplatin bearing poly (epsilon-caprolactone) nanoparticles amalgamated with in situ gel tendered augmented drug delivery, cytotoxicity, and apoptosis in melanoma tumor. Colloids Surf B Biointerfaces. 2018;166:339–48.
Hidalgo Pitaluga L, Trevelin Souza M, Dutra Zanotto E, Santocildes Romero ME, Hatton PV. Electrospun F18 bioactive glass/PCL-Poly (epsilon-caprolactone)-membrane for guided tissue regeneration. Materials (Basel), 2018;11. http://www.ncbi.nlm.nih.gov/pubmed/29517988.
Kock L, van Donkelaar CC, Ito K. Tissue engineering of functional articular cartilage: the current status. Cell Tissue Res. 2012;347:613–27.
Drury JL, Mooney DJ. Hydrogels for tissue engineering: scaffold design variables and applications. Biomaterials. 2003;24:4337–51.
Huey DJ, Hu JC, Athanasiou KA. Unlike bone, cartilage regeneration remains elusive. Science. 2012;338:917–21.
Johnstone B, Yoo JU. Autologous mesenchymal progenitor cells in articular cartilage repair. Clin Orthop Relat Res. 1999(367 Suppl): S156–62, http://www.ncbi.nlm.nih.gov/pubmed/10546644.
Engelmayr GCJr, Cheng M, Bettinger CJ, Borenstein JT, Langer R, Freed LE. Accordion-like honeycombs for tissue engineering of cardiac anisotropy. Nat Mater. 2008;7:1003–10.
Liu T, Mu H, Shen Z, Song Z, Chen X, Wang Y. Autologous adipose tissue derived mesenchymal stem cells are involved in rat liver regeneration following repeat partial hepatectomy. Mol Med Rep. 2016;13:2053–9.
Greenstein G, Caton JG. Biodegradable barriers and guided tissue regeneration. Periodontol 2000. 1993;1:36–45.
Duan P, Pan Z, Cao L, He Y, Wang H, Qu Z. et al. The effects of pore size in bilayered poly(lactide-co-glycolide) scaffolds on restoring osteochondral defects in rabbits. J Biomed Mater Res A. 2014;102:180–92.
Bruckner P, van der Rest M. Structure and function of cartilage collagens. Microsc Res Tech. 1994;28:378–84.
Acknowledgements
This work was supported by the National Natural Science Foundation of China (No. 81371706, No. 81201212), and the National Natural Science Foundation of China (No. 81601901).
Author information
Authors and Affiliations
Corresponding author
Ethics declarations
Conflict of interest
The authors declare that they have no conflict of interest.
Additional information
Publisher’s note: Springer Nature remains neutral with regard to jurisdictional claims in published maps and institutional affiliations.
Rights and permissions
About this article
Cite this article
Liu, Y., Tian, K., Hao, J. et al. Biomimetic poly(glycerol sebacate)/polycaprolactone blend scaffolds for cartilage tissue engineering. J Mater Sci: Mater Med 30, 53 (2019). https://doi.org/10.1007/s10856-019-6257-3
Received:
Accepted:
Published:
DOI: https://doi.org/10.1007/s10856-019-6257-3