Abstract
A combined theoretical and experimental approach is developed for determining the local efficiency of multicrystalline silicon solar cells by use of quantitative lock-in carrierographic (LIC, also described as frequency-domain photoluminescence, LIP) and lock-in thermographic (LIT) imaging. The characteristic distribution of the saturation current density \(J_{0}\), open-circuit voltage \(V_{\mathrm{OC}}\), fill factor FF, and efficiency of solar cell \(\eta _{\mathrm{s}}\) are obtained, and the local inhomogeneities of a solar cell are readily determined by LIT and LIC imaging. The combination of a quantitative LIT and LIC imaging approach is a valuable tool for judging which local defects are especially harmful for degrading the fill factor or the open-circuit voltage.
Similar content being viewed by others
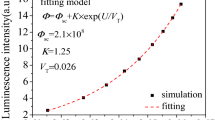
Avoid common mistakes on your manuscript.
1 Introduction
The current–voltage characteristic plays an important role in estimating the performance of solar cells. It directly determines the quality and conversion efficiency. The current–voltage characteristic can be described by the electrical fundamental parameters of the solar cell. Over the past two to three decades, many infrared radiometric methods have been used to evaluate the performance of solar cells. They mainly include photothermal radiometry (PTR), lock-in thermography (LIT), photoluminescence imaging (PL), photocarrier radiometry (PCR), and lock-in carrierography (LIC), among others [1–5].
Lock-in thermography (LIT), which includes dark lock-in thermography (DLIT) and illumination lock-in thermography (ILIT), is a useful characterization imaging technique widely used in solar cell research [6–9]. LIT is available for detection of shunts, series resistance, and grain boundaries, and can be used to determine the power loss based on an appropriate calibration.
Long-pass-filtered dynamic lock-in photoluminescence imaging (LIPI), also known as lock-in carrierography (LIC) imaging, was recently developed [10] as the dynamic imaging extension of photocarrier radiometry (PCR) [11]. It has the advantage of filtering out the thermal infrared (non-radiative) component of the emission spectrum of de-exciting free photocarriers, and/or resolving the radiative versus the non-radiative components through spectrally windowed modulation frequency scans, thereby being sensitive only to radiative recombination events when performing dynamic measurements of transport properties in semiconductors [12].
In this paper, the relation between the LIT amplitude, power loss flux, and a quantitative calibration expression for the LIT amplitude is described. A quantitative relation [14, 15] that links the radiative recombination emission flux to the electrical parameters of solar cells is used for the determination of the same fundamental parameters by means of LIC measurements. Furthermore, both LIT and LIC are used to determine the local efficiency of a solar cell.
2 Experimental Apparatus and Procedures
2.1 Samples
An industrial multicrystalline-Si (mc-Si) solar cell (\(156\times 156\,\hbox {mm}^{2}\), 0.2 mm thickness) from Enfoton Solar Ltd., Cyprus, was used for both LIT and LIC measurements.
2.2 Description of Experimental Technique
2.2.1 Principle of the Technique
Non-thermal radiation, as encountered in semiconductors under nonequilibrium conditions, has been described with the generalization of Planck’s law as introduced by Würfel [13]. For optoelectronic semiconductor materials of bandgap energy \(E>1\, \hbox {eV}\), and small photovoltages such that \(E- qV \ll k_{\mathrm{B}}T\), where \(q\) is the elementary charge and \(k_{\mathrm{B}}T\) is the thermal energy, the emitted photon flux can be accurately simplified as
\(\eta _{\mathrm{ce}} [{\hbar {\omega }} ,V({\hbar {\omega }} ),T]_{x,y} \) is defined to be the carrier collection efficiency, the ratio of the collected carrier flux to the photogenerated carrier flux [14–16],
Quantum efficiencies for non-radiative and radiative recombination, \(\eta _{\mathrm{NR}}\) and \(\eta _{\mathrm{R}}\), are given at open-circuit [14–16] conditions,
The photogenerated current density (bias voltage \(V ({\hbar {\omega }})=0\)) is given by [14]
The dissipated power flux \(E_{\mathrm{loss}}\) of a solar cell under monochromatic illumination is related to the photogenerated current density \(J_{\mathrm{g}}\), the injection current density \(J_{\mathrm{D}}\), the photovoltage \(V\) (\({\hbar {\omega }}\)), and the photon energy \({\hbar {\omega }}_{\mathrm{in}}\) [1–4]:
Furthermore, the injection current density depends exponentially on the photovoltage,
It should be noted that for dynamic imaging modalities at an angular modulation frequency \(\omega _{\mathrm{M}}\), such as in LIT and LIC imaging, complex quantities are implied with the general form, \(Z({\hbar {\omega }} )=\left| {Z({\hbar {\omega }} )} \right| {\cdot }\hbox {e}^{\mathrm{i}\phi ({\hbar {\omega }} )}{\cdot }\hbox {e}^{\mathrm{i}\omega _\mathrm{M} t}\). The LIT amplitude is proportional to the dissipated power, and the proportionality factor can be identified as a calibration factor, \(C_{\mathrm{LIT}}\) [3],
The calibration factor, \(C_{\mathrm{LIT}}\), is given by [14]
From Eqs. 5 and 7, an expression for the LIT amplitude related to the electrical parameters of the solar cell can be derived as
where \(A_0 =C_\mathrm{LIT} (x,y)J_0 (x,y), A_\mathrm{g} =C_\mathrm{LIT} (x,y)J_\mathrm{g} (x,y)\), and \(C=\frac{{\hbar {\omega }} _{\mathrm{in}} }{q\eta \eta _{\mathrm{ce}} [{\hbar {\omega }} ,0,T]_{x,y} }\).
From Eq. 9, the electrical fundamental parameters of a solar cell can be accurately estimated by means of LIT measurements.
In LIC, the influence of the excess minority carrier reabsorption is negligible [10], as the absorption efficiency for radiatively emitted luminescence photons is small. Introduction of an optoelectronic ideality factor \(n_{j}\) describing radiative recombination processes, an equation has been derived that is only related to the nonequilibrium radiative recombination process [14, 15],
where \(J_\mathrm{R} (x,y)=q{\cdot } F_\mathrm{R} ({\hbar {\omega }} ,V_{\mathrm{OC}} ,T)_{x,y}\) and \(J_{\mathrm{R0}} (x,y)=qF_\mathrm{R} (0,T)_{x,y} \).
The calibration factor and photogenerated current density are given by [14, 15]
and
A number of expressions can be derived. The most relevant quantities are the open-circuit photovoltage relationship,
and an expression for the fill factor (FF) has been provided by Ghosh et al. [17],
The solar cell efficiency is obtained from
2.2.2 Apparatus
For the LIT and LIC measurement setup made as shown in Fig. 1a, the optical-to-photocarrier flux can be described according to Fig. 1b. A mid-infrared camera (Cedip Titanium 550 from FLIR) with a \(320\times 256\) pixel active element, spectral bandwidth of \(2.5\,{\upmu }\hbox {m}\) to \(5.0\,{\upmu }\hbox {m}\), and frame rate of 150 Hz for a full window size was used for LIT measurements. A near-infrared camera (SU320KTSW-1, 7RT/RS170 from Goodrich Sensors Unlimited) with a long-pass filter (\({\sim }1000\,\hbox {nm}\)) was used for LIC measurements. This camera has a \(320\times 256\) pixel active element, spectral bandwidth of \(0.9\,{\upmu }\hbox {m}\) to \(1.7\,{\upmu }\hbox {m}\), frame rate of 119.6 Hz for full window size, and full-frame exposure times ranging from 0.13 ms to 16.6 ms. The solar cell was illuminated from the front side using a superband-gap fiber coupled 16 W/808 nm diode laser. The laser beam was spread and homogenized by engineered microlens arrays forming a square illumination area with a uniform intensity. A data acquisition module from National Instruments was used to generate the modulation waveform for the laser current. The modulation frequency was set at 10 Hz, and the LIC and LIT amplitude and phase images were produced using in-house developed software.
A series of load resistances were used to measure the I–V characteristics of our solar cell with dc illumination provided by the same 808 nm diode laser under the same conditions. The current \(I\) through the load resistance \(R_{\mathrm{L}}\) and the photovoltage \(V\) across \(R_{\mathrm{L}}\) were obtained from electrical measurements (EM).
3 Results
The LIT and LIC experiments were carried out in two different experimental systems, and the light intensity was set as close as possible to \(0.04\,\hbox {W}{\cdot }\hbox {cm}^{-2}\). In practice, the illumination intensity was measured to be \(0.043\,\hbox {W}{\cdot }\hbox {cm}^{-2}\) and \(0.038\,\hbox {W}{\cdot }\hbox {cm}^{-2}\) for the LIT and LIC measurements, respectively. The \(I{-}V\) characteristic, power output versus photovoltage curve and their theoretical best fits to the solar cell diode equation without a series resistance are shown in Fig. 2. The photovoltage corresponding to maximum power, \(V_{\mathrm{pmax}}=0.462\,\hbox {V}\) (\(I_{\mathrm{i}}=0.043\,\hbox {W}{\cdot }\hbox {cm}^{-2})\) and \(V_{\mathrm{pmax}}=0.460\,\hbox {V}\) (\(I_{\mathrm{i}}=0.038\,\hbox {W}{\cdot }\hbox {cm}^{-2})\) was obtained from the peak of the fitted curve in Fig. 2b. The fill factor and solar efficiency were calculated using FF \( =P_{\mathrm{max}}/V_{\mathrm{oc}}J_{\mathrm{sc}}S\) and \(\eta _{\mathrm{s}}= P_{\mathrm{max}}/I_{\mathrm{i}}{\cdot } S\), respectively. \(\textit{FF}=72.14 \%, \eta _{\mathrm{s}}=18.3\%\) (\(I_{\mathrm{i}}=0.043\,\hbox {W}{\cdot }\hbox {cm}^{-2})\) and \(\textit{FF}=72.24 \%, \eta =18.6\%\) (\(I_{\mathrm{i}}=0.038\,\hbox {W}{\cdot }\hbox {cm}^{-2})\) were also obtained from Fig. 2. Therefore, it indicates that the electrical performance (FF and \(\eta _{\mathrm{s}})\) of the solar cell is fully similar for two different light intensities.
To validate the proportionality between the LIT amplitude and power dissipation, the voltage-dependent LIT measurements are shown in Fig. 3. The calibration factor based on non-radiative recombination according to Eq. 8 was used to calibrate the LIT amplitude image dependence on power losses. The total power dissipated in the whole solar cell for every voltage setting was determined from the LIT measurements by integration over the entire solar cell area. By adding the electrically extracted power to the dissipated power measured by LIT, we obtained a variable power component over the entire voltage range as seen in Fig. 3. This power component is less than the irradiation power incident into the cell. This indicates that a small fraction of the irradiation power is reflected by the solar cell surface as well as emitted by photocarrier radiative recombination after absorption. The LIT-calculated photovoltage corresponding to maximum power \(V_{\mathrm{pmax}}=0.464\,\hbox {V}\) was obtained at the minimum value of the dissipated power in Fig. 3.
Figure 4 shows saturation diode current density \(J_{0}\) images of the solar cell calculated as derivative images from LIT and LIC images. The \(J_{0}\) images, scaled in \({\upmu }\hbox {A}{\cdot }\hbox {cm}^{-2}\), are shown in Fig. 4a and b using the LIT and LIC images, respectively. From the LIT image, Fig. 4a, the local saturation diode current density \(J_{0}\) of the solar cell is seen to vary between \(0.1\,{\upmu }\hbox {A}{\cdot } \hbox {cm}^{-2}\) to \(0.2\,{\upmu }\hbox {A}{\cdot }\hbox {cm}^{-2}\), and local inhomogeneities are apparent with high contrast. From the LIC image, Fig. 4b, the local saturation diode density, \(J_{0}\), of the solar cell is seen to vary between \(0.05\,{\upmu }\hbox {A}{\cdot }\hbox {cm}^{-2}\) to \(0.5\,{\upmu }\hbox {A}{\cdot }\hbox {cm}^{-2}\), and local inhomogeneities are also apparent with high contrast. The images agree with the theoretical expectation that a high local concentration of recombination centers crossing the \(p-n\) junction leads to pronounced enhanced recombination which is responsible for the high saturation diode current density and/or high ideality factors [8].
In Fig. 5 various local cell parameters are shown using LIT and LIC imaging, which are obtained assuming full illumination intensity (\(I_{\mathrm{i}}=0.043\,\hbox {W}{\cdot }\hbox {cm}^{-2}\) for LIT imaging; and \(I_{\mathrm{i}}=0.038\,\hbox {W}{\cdot }\hbox {cm}^{-2}\) for LIC imaging). It is seen that the LIT \(V_{\mathrm{OC}}\) at \(0.043\,\hbox {W}{\cdot }\hbox {cm}^{-2}\) and the LIC \(V_{\mathrm{OC}}\) at \(0.038\,\hbox {W}{\cdot }\hbox {cm}^{-2}\) (from Fig. 5a and d, respectively) are mainly influenced by local maxima of the saturation diode current density (in Fig. 4). The opposite holds for the FF distributions in Fig. 5b and e, respectively, which are more strongly influenced by the maxima of the saturation diode current density. All local efficiency variations (Fig. 5c and f, respectively) may be explained by variations of \(V_{\mathrm{OC}}\) or FF. The local solar cell efficiency analysis using LIT and LIC measurements is consistent with earlier results [8]. From Figs. 4 and 5, the saturation diode current density, \(J_{0}\), and the FF of the LIC measurements exhibit a higher dynamic range than the respective LIT measurements. It can be seen that the LIC measurements are more sensitive to local inhomogeneities than the LIT measurements, and the LIC-derived \(V_{\mathrm{OC}}\), FF, and \(\eta \) images show high spatial resolution and contrast.
(a) LIT-\(V_{\mathrm{OC}}\) at \(0.043\,\hbox {W}{\cdot }\hbox {cm}^{-2}\), (b) LIT-FF at \(0.043\,\hbox {W}{\cdot }\hbox {cm}^{-2}\), (c) LIT-\(\eta _{\mathrm{s}}\) at \(0.043\,\hbox {W}{\cdot }\hbox {cm}^{-2}\), (d) LIC-\(V_{\mathrm{OC}}\) at \(0.038\,\hbox {W}{\cdot }\hbox {cm}^{-2}\), (e) LIC-FF at \(0.038\,\hbox {W}{\cdot }\hbox {cm}^{-2}\), and (f) LIC-\(\eta _{\mathrm{s}}\) at \(0.038\,\hbox {W}{\cdot }\hbox {cm}^{-2}\). All scaling ranges are indicated and scaling bars in (d) hold for all images
4 Conclusions
We have demonstrated that quantitative LIT and LIC imaging can be used to analyze the local inhomogeneities and local efficiencies of solar cells. LIC has the advantage of higher spatial resolution and contrast, including the saturation diode current density \(J_{0}\), open-circuit voltage \(V_{\mathrm{OC}}\), fill factor FF, and efficiency \(\eta _{\mathrm{s}}\) images. The combination of quantitative LIT and LIP imaging can be a more valuable tool for judging which local defects are especially harmful for degrading the fill factor or the open-circuit voltage than either technique alone.
Abbreviations
- \(A_0 =C_{\mathrm{LIT}} J_0 , A_\mathrm{g} =C_{\mathrm{LIT}} J_\mathrm{g}, C=\frac{{\hbar {\omega }} _{\mathrm{in}} }{q\eta \eta _{\mathrm{ce}} ({\hbar {\omega }} ,0,T)}\) :
-
Intermediate parameters
- \(C_{\mathrm{LIT}}, C_{\mathrm{LIC}}\) :
-
Calibration factor of LIT and LIC
- \(F_{\mathrm{R}}(V,T)\) :
-
Radiative-recombination emission photon flux
- \(F_{i} ({\hbar {\omega }} )\) :
-
Incident photon flux
- \(F_{\mathrm{ce}} {[}{{\hbar {\omega }}} ,V({\hbar {\omega }} ),T{]}\) :
-
Photocarrier flux collected at the \(p{-}n\) junction
- FF :
-
Fill factor
- \(\left| {\hbox {LIT}[V(\omega _\mathrm{M} )]} \right| _{x,y} , \left| {\hbox {LIC}[V(\omega _\mathrm{M} )]} \right| _{x,y}\) :
-
LIT amplitude and LIC amplitude distribution
- \(J_{\mathrm{g}}, J_{\mathrm{D}}, J_{0}\) :
-
Photogenerated current density, injection current density, and saturation diode current density
- \(J{[}{\hbar {\omega }},V({\hbar {\omega }}\omega ),T{]}_{R}\) :
-
Nonequilibrium radiative recombination current density
- \(J_\mathrm{R} =q{\cdot }\eta \eta _\mathrm{R} ({\hbar {\omega }} ,V_{\mathrm{OC}} ,T)F_i ({\hbar {\omega }} ) ,J_{\mathrm{R0}} =qF_\mathrm{R} (0,T)\) :
-
Relevant current-like quantities
- \({\vert }I_{i}{\vert }\) :
-
Peak value of incident modulation illumination intensity
- \(R\) :
-
Surface reflectance
- \(S\) :
-
Illuminated surface area of the solar cell
- \(V_{\mathrm{OC}}\) :
-
Open-circuit voltage
- \(Z({\hbar {\omega }} )=\left| {Z({\hbar {\omega }} )} \right| {\cdot }\hbox {e}^{i\phi ({\hbar {\omega }} )}{\cdot } \hbox {e}^{\mathrm{i}\omega _\mathrm{M} t}\) :
-
Complex quantities
- \(n, n_{j}\) :
-
Ideality factor of solar cell and optoelectronic ideality factor
- \(\alpha (E)\) :
-
Absorptivity of an emitter of non-thermal radiation
- \(\eta , \eta _\mathrm{ce}\) :
-
Quantum efficiency and photocarrier-to-collection current efficiency
- \(\eta _{\mathrm{s}}\) :
-
Efficiency of solar cell
- \({\hbar {\omega }} _{\mathrm{in}} , {\hbar {\omega }} _{\mathrm{em}} \) :
-
Incident and mean radiative-recombination-emission photon energy
- \(\eta _{\mathrm{NR}}, \eta _{\mathrm{R}}\) :
-
Quantum efficiency for non-radiative and radiative recombination
- \(\lambda _{\mathrm{in}}, \lambda _{\mathrm{em}}\) :
-
Photon wavelengths corresponding to \({\hbar {\omega }} _{\mathrm{in}} \) and \({\hbar {\omega }} _{\mathrm{em}} \)
References
D. Cahen, B. Büchner, P. Decker, M. Wolf, IEEE Trans. Electron Dev. 37, 498 (1990)
H. Flaisher, M. Wolf, D. Cahen, J. Appl. Phys. 66, 1832 (1989)
M. Wolf, D. Cahen, Solar Cells 27, 247 (1989)
D. Cahen, Appl. Phys. Lett. 33, 810 (1978)
A. Mandelis, J. Appl. Phys. 66, 5572 (1989)
O. Breitenstein, M. Langenkamp, M.H.A. Rifai, Prog. Photovolt. Res. Appl. 11, 515 (2003)
O. Breitenstein, M. Langenkamp, O. Lang, A. Schirrmacher, Sol. Energy Mater. Sol. Cells 65, 55 (2001)
O. Breitenstein, Sol. Energy Mater. Sol. Cells 107, 381 (2012)
O. Breitenstein, J.P. Rakotoniaina, J. Appl. Phys. 97, 074905 (2005)
A. Melnikov, A. Mandelis, J. Tolev, P. Chen, S. Huq, J. Appl. Phys. 107, 114513 (2010)
A. Mandelis, J. Batista, D. Shaughnessy, Phys. Rev. B 67, 205208 (2003)
A. Melnikov, A. Mandelis, J. Tolev, E. Lioudakis, J. Phys. Conf. Ser. 214, 012111 (2010)
P. Würfel, J. Phys. C 15, 3967 (1982)
J.Y. Liu, A. Melnikov, A. Mandelis, Phys. Status Solidi A 210, 2135 (2013)
J.Y. Liu, A. Melnikov, A. Mandelis, J. Appl. Phys. 114, 104509 (2013)
A. Mandelis, Y. Zhang, A. Melnikov, J. Appl. Phys. 112, 054505 (2012)
A.K. Ghosh, C. Fishman, T. Teng, J. Appl. Phys. 51, 446 (1980)
Acknowledgments
A. Mandelis is grateful to NSERC for a Discovery grant, to the Canada Foundation for Innovation (CFI) for equipment grants, to the Canada Research Chairs Program, and to the Ontario Ministry for Research and Innovation (MRI) for the Inaugural Premier’s Discovery Award in Science and Technology (2007).
Author information
Authors and Affiliations
Corresponding author
Rights and permissions
About this article
Cite this article
Liu, J.Y., Melnikov, A. & Mandelis, A. Non-contact Determination of Local Efficiency of mc-Si Solar Cells Using Quantitative Lock-In Thermographic and Carrierographic (Photoluminescence) Imaging. Int J Thermophys 36, 987–996 (2015). https://doi.org/10.1007/s10765-014-1786-y
Received:
Accepted:
Published:
Issue Date:
DOI: https://doi.org/10.1007/s10765-014-1786-y