Abstract
Aerosolized microorganisms have become an important factor in assessing air quality. To determine the characteristics of bacterial bioaerosols in air and rainwater, as well as calculate the recovery rate of bacteria after rains in Ho Chi Minh City, our study was performed using the SKC Biostage sampler for airborne bacteria and Plate Count Agar (PCA) medium for bacterial concentration. Subsequently, the study determined the bacterial community composition at the phylum and order levels using the 16S rRNA (16S metabarcoding) method. Before the rain, bacterial concentrations in the air ranged from 263.39 ± 21.00 to 277.39 ± 78.99 CFU/m3, and in rainwater 264.89 ± 51.17 to 285.72 ± 28.00 CFU/m3. Following rains, the bacterial concentrations decreased to their lowest levels within the first 1–2 h and gradually increased thereafter, reaching their peak after 9 h for heavy rain and after 12 h for light and moderate rains. The bacterial bioaerosols recovery rate was determined to be 100% for light and moderate rains and 94.6% for heavy rain. The change in bacterial concentration after rainfall showed a positive correlation with temperature (r = 0.85) and CO2 concentration (r = 0.70) and a negative correlation with relative humidity (r = − 0.79). Bacterial composition analysis revealed that the Actinobacteria, Firmicutes, and Proteobacteria phyla were dominant and characteristic of the humid tropical climate in Vietnam. Notably, Firmicutes were the most prevalent phylum both before and after rains. The increased prevalence of certain bacterial orders, particularly Staphylococcus, could contribute to the spread of pathogens, particularly foodborne pathogens. In addition to rain, relative humidity contributed to reducing bacterial bioaerosols concentration and their recovery rate after the rain.
Similar content being viewed by others
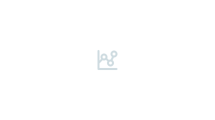
Explore related subjects
Discover the latest articles, news and stories from top researchers in related subjects.Avoid common mistakes on your manuscript.
Introduction
Bioaerosols are tiny particles in the air, ranging from 0.3 to 100 µm in diameter. They consist of biological fragments from plants, animals, and active organisms like bacteria, viruses, and fungi (Georgakopoulos et al., 2009; Stetzenbach, 2009). These particles exist in soil, water, and air environments, varying in types, compositions, and sizes. Among microorganisms, bacteria are particularly abundant and contribute to the characterization of bioaerosols under specific weather and geographical conditions. In the atmosphere, microorganisms can be attached to other particles or be incorporated into water droplets like fog or raindrops. Through advection processes, they can be transported from one environment to another (Polymenakou, 2012). Aerosolized microorganisms possess properties that have a significant impact on altering the composition and function of aquatic and terrestrial microbiota. Additionally, they play a crucial role in climate change, soil–water pollution, and disease transmission (Joung et al., 2017). Some well-known examples of airborne diseases include pneumonia, tuberculosis, brucellosis, anthrax, and Q fever. These diseases pose a threat to public health and adversely affect the community’s quality of life within communities (Arancibia et al., 2002).
The density and diversity of bacteria present in the air are observed to differ with the changing seasons. This variation is majorly influenced by the source of microbial dispersal and the prevailing weather conditions in each season (Zhen et al., 2017). Furthermore, human activities contribute to fluctuations in air pollutants (such as CO2, O3, and SO2), which are also considered factors affecting the survival of microorganisms (Dong et al., 2016). The occurrence of rains results in a sudden change not only in the concentration of bacteria in the air but also in their diversity via rainwater. Microorganisms attach themselves to airborne particles, which are then captured in raindrops and fall to the ground (Smets et al., 2016). This process may be the reason why the sky appears bluer and clearer after rains in areas with high pollution as observed by Polymenakou (2012).
Bacteria can be added to the atmosphere in several ways, such as through wind, air convection currents from the soil, or through rain, water droplets splashing up at the ground–air interface that will transfer the microorganisms to the atmosphere (Joung et al., 2017). Some studies have shown that rain has a washing effect and leads to a decrease in bacterial concentrations compared to concentrations before the rain (Joung et al., 2017; Kulshrestha et al., 2009). However, there are increasing studies showing the opposite result, precipitation events enhancing concentrations of bacteria (Kang et al., 2015; Seinfeld & Pandis, 2006). The timing of sampling after the rain may explain the difference in these findings, suggesting the need to monitor the bacterial aerosol concentration at different periods after the rain. Moreover, an increase in the concentration of bacteria in the air after the rain has the potential to increase the occurrence of some pathogens due to the disturbance of the microbiome (Joung et al., 2017). Therefore, understanding the microbial composition, rate, and recovery time (concentration) of bacteria in the air after rains is crucial for strategies to protect public health, especially in cities and countries with high pollution.
Vietnam is a country in Southeast Asia, with both tropical and temperate climates, influenced by the annual monsoon, bringing a relatively large average annual rainfall of about 1766.84 mm (CCKP, 2021). However, air pollution in big cities is currently at an alarming level, with the air quality index (AQI) in the moderate range (AQI, 2023), significantly impacting the community’s health and quality of life. According to WHO statistics, every year Vietnam has about 60,000 deaths related to air pollution (WHO, 2023). The effect of rains on the increase in bacterial population has been shown to raise great concern about the potential development of pathogens after each rain for the population in this country. However, studies on the composition and density of bacteria before and after rains as well as the resilience of microorganisms after rains in Southeast Asia, especially Vietnam, are still very limited (Fang et al., 2005; Hu et al., 2017; Wang et al., 2010; Wiśniewska et al., 2022).
Metabarcoding of the 16S rRNA gene is a valuable tool for comprehending the variety of microbial populations in the atmosphere both before and after rainfall. This helps in making impartial evaluations of their impact on ecology, climate, and public health (Hu et al., 2017; Kaushik et al., 2014; Peter et al., 2014). Herein, we aim to study the impact of rainfall and meteorological conditions on the atmospheric microbial communities at a location in Ho Chi Minh City. The 16S rRNA metabarcoding was employed to characterize the culturable microbial compositions in aerosol and rainwater samples. The study comprises two major objectives: (i) characterize the changes in density and compositional structure of culturable bacteria in rainwater and in aerosol samples before and after rainfall and (ii) investigate the association of these changes with meteorological conditions.
Materials and methods
Sample collection
The sample collection process of the entire experiment took place during the period from September to November 2022 at the University of Natural Sciences, Vietnam National University, Ho Chi Minh City. Rainwater sampling was performed on the roof of the University’s building E located at coordinates 10° 45′ 46.3″ N and 106° 40′ 54.4″E using a rainwater sampler including a rainwater container, a sensor for measuring rainwater, and rainwater-connecting data system (HOBO Data Loggers). Before each sample collection (Lonon, 1998), the sampler was thoroughly cleaned with soap, warm and cold tap water, 10% HCl solution, and finally rinsed with Milli-Q water.
Bioaerosol samples were collected near the rainwater sampling site, specifically at the main entrance of the university. This area is surrounded by trees and is approximately 10 m away from a major traffic route with frequent student activity (Fig. 1). Aerosol bacterial sampling was conducted using a culture method on Plate Count Agar (PCA) plates. A petri dish containing 15 mL PCA was placed in an SKC Biostage aerosol collector (SKC, USA) equipped with a pump following the guidelines of the manufacturer’s instructions. Sampling was conducted before the rain (approximately 1–2 h prior) and then hourly for 24 h after the rain. There were also blank samplings serving as negative controls. The sampling time has a 5-min duration and a flow rate of 28.3 L/min (Lonon, 1998). The collection point was 1.5 m above the ground. Before each sampling, the SKC Biostage equipment was meticulously cleaned by disassembling it, immersing the components in an ultrasonic bath filled with Mili-Q water, and disinfecting the sampling site using a sterile swab soaked in 70% ethanol. After sampling, all collected samples (both bioaerosol and rainwater) were promptly transported to the laboratory for processing and subsequent experiments. Meteorological parameters such as temperature, relative humidity, CO2, and PM2.5 (data not shown) were continuously recorded at the biological dust sampling site using the Aerobox outdoor Sensor kit (Plantower PMS7003), National Central University (NCU), Taiwan, by the PM2.5@Asia project. The sensor kit was calibrated 6 months per time by the research team of NCU University, Taiwan. Precipitation parameter was recorded by a HOBO data logging rain gauge (www.onsetcomp.com) (He et al., 2018). Besides, wind speed (WS) and solar radiation (SR) were followed by the website AccuWeather Ho Chi Minh City (AccuWeather, 2023).
Determination of bacteria concentration
For bioaerosol samples, PCA agar plates that had been exposed to the air were removed from the sample collector, marked with each time point (each time point corresponding to two plates, n = 2), and covered under aseptic conditions. The collected rainwater samples were diluted to the appropriate concentration; then, 100 µL of the samples were quickly spread onto agar plates containing nutrient agar (NA) medium using a sterilized glass spreader, with two replicates (n = 2). We then incubated all plates at 37 ± 1 °C for 24 h (FAO, 1991). Formed colonies were counted, and the colony-forming units of bioaerosols C were determined using the following formula (1) (Malakootian & Gharghani, 2016).
where P is the number of colonies grown in the plate (CFU), 1000 is the conversion factor from liters to cubic meters (m3) (volume of air), T is the sampling time (min), and Q is the sampling air flow (L/min).
Bacterial concentration in rainwater samples was calculated from the number of colonies growing on the plate corresponding to the dilution used for each plate and converted as described in formulas (2.1) and (2.2), respectively.
where P is the number of colonies grown in the plate (CFU), d is the dilution ratio, and 106 is the conversion factor from milliliters to cubic meters (volume of rainwater).
Recovery rate of bioaerosol bacteria
To evaluate the recovery ability of the cultured aerosol bacterial community after rainfall, the study determined the recovery rate of bioaerosol bacteria. In the present study, recovery rate was defined as the percentage of the highest post-rain aerosol bacterial concentration compared to the pre-rain bacterial concentration. Before being used to calculate recovery rates, pre-rain, and post-rain values were tested for statistically significant differences (p < 0.05).
DNA extraction and 16S rRNA metabarcoding
All formed colonies on the agar plates were collected for DNA extraction. The DNA was extracted using the Dneasy PowerSoil Pro kit (Qiagen, USA) following the manufacturer’s instructions and stored at − 20 °C for further analysis. The quantity and quality of extracted DNAs were determined by the photometric method (Qubit). The OD260/OD280 values were determined by optical density measurement. Samples were considered to be of high quality for the next steps when the concentration was ≥ 0.20 ng/µL and the OD260/OD280 value was ≥ 1.70. Qualified samples were then amplified by PCR of the V1-V9 region of the bacterial 16S rRNA gene. The primer mixture for multiplex PCR was in the Library Prep with product code IDT xGen™ 16S Amplicon Panel v2 (96RXN) and Cat.No. 10009828. The 16S rRNA sequencing library was prepared with the xGen 16S Amplicon Panel v2 kit (IDT, USA) following the manufacturer’s instructions. Enzymes were used xGen Normalase™ reagents. Pre-program the thermal cycler for multiplex PCR and allow the block to reach 98 °C before loading the samples. Library concentration was determined by the qPCR method according to the instructions of the KAPA Library Quantification Kit Illumina® Platforms kit (Cat. No. KR0405). The concentration of samples was ≥ 0.30 nM and was considered to be sufficient quality for sequencing. The library was then sequenced using the 150PE next-generation sequencing method on a MiniSeq instrument. The optimal number of reads for analysis was 100,000 read pairs per sample.
Low-quality sequencing reads, adapters, and primers were filtered out and trimmed by Trimmomatic version 0.39 (Bolger et al., 2014) and Cutadapt version 2.10 (Martin, 2011). The proportions of high-quality sequencing reads remaining for further analysis were between 270,000 and 360,000 for all samples. Amplicon sequence variants (ASVs) were then generated from the processed reads and denoised with QIIME2 version 2021.11.0 (Bolyen et al., 2019) and the q2-DADA2 plugin (Callahan et al., 2016). Taxonomy assignment was performed on the ASVs using the SILVA database version 138 SSURef Nr99 (Quast et al., 2013) and the q2-feature-classifier plugin and classify-consensus-blast method of QIIME2 version 2021.11.0 (Bolyen et al., 2019). Rarefaction curves were constructed based on observed richness values computed by QIIME2 (Bolyen et al., 2019). Plateau was reached in the rarefaction curves of all samples, indicating that the data quantities were sufficient for community diversity assessment. All analyses were conducted using default parameters.
Statistical analysis
In our study, Spearman’s correlation coefficient was used to assess the impact of meteorological factors (including temperature, relative humidity, and concentration of CO2) on bacterial concentration. Statistical analysis was performed to compare aerosol bacterial concentrations before and after the rain and aerosol concentrations at a time between the three types of rains using the Mann–Whitney U-test and Kruskal–Wallis test, respectively. The results for aerosolized bacteria concentrations were presented as mean ± SD.
Results and discussion
Meteorological conditions during sampling
Rain classification is based on rainfall rate (mm/h) as described by the World Meteorological Organization (World Meteorological Organization, 2018). Light rains in the study were defined as rains with rainfalls of 0.8 and 2.8 mm/h recorded on October 25–26 and September 25–26, respectively. Moderate rains with rainfalls of 9.08 to 12.2 mm/h occurred on November 23–24 and October 27–28. Finally, heavy rains with rainfalls of 26.5 and 28.3 mm/h were recorded on November 3–4 and September 29–30. Figure 2 shows the variation trend of aerosol bacteria concentration along with temperature (a), relative humidity (b), and CO2 concentration (c) according to the rain event (September to November 2022). Temperatures during the 3 months of sampling ranged from 24.1 to 32.8 °C, relative humidity ranged from 54.8 to 93.2%, and CO2 concentrations ranged from 332 to 864 ppm.
The correlation between the concentration of bacterial bioaerosols with temperature, relative humidity, and CO2 concentration was analyzed. The correlation coefficient between average bacterial concentration and temperature, CO2 concentration, and relative humidity was 0.85 (p < 0.001), 0.70 (p < 0.001), and − 0.79 (p < 0.001), respectively.
The effect of rainfall on bacterial resilience after the rain
The variation in bacterial concentration under different rain conditions (light, moderate, and heavy rains) was investigated and presented in Table 1 and Fig. 3. The recovery rate of bacteria, in terms of bacterial concentration, was also calculated and shown in Table 1.
Prior to the rain, there was little variation in bacterial concentration among the three types of rain (p > 0.05). The values were as follows: 277.39 ± 78.99 (CFU/m3) for light rain, 274.00 ± 31.00 for moderate rain, and 263.39 ± 21.00 for heavy rain. In rainwater samples, the bacterial concentration was recorded as 264.89 ± 51.17, 285.72 ± 28.00, and 271.54 ± 118.00 (CFU/m3) for light, moderate, and heavy rains, respectively. Interestingly, the concentration of bacteria in moderate rain samples was higher than in light and heavy rain samples.
The change in bacterial concentration for each type of rain revealed slight differences. The bacterial concentration of heavy rain in the first hour after the rain was 105.67 ± 61.74 CFU/m3, reaching its highest at 9 h (249.12 ± 31.27 CFU/m3) during the 24-h period after the rain. The recovery rate for bacterial concentration was calculated as 94.6%. Afterward, the bacterial concentration gradually decreased and reached a low level in the next 12 h. Light and moderate rain showed a significant reduction in aerosol bacterial concentrations in the initial hours after the rain. The lowest bacterial concentrations were observed 2 h after light rain (93.64 ± 15.67 CFU/m3) and 1 h after moderate rain (112.45 ± 127.00 CFU/m3). Subsequently, the bacterial concentration gradually increased and peaked at 12 h after the rain (295.05 ± 19.46 CFU/m3 for light rain and 277.39 ± 17.67 CFU/m3 for moderate rain). The recovery rate was high for both light and moderate rains. Following the peak, the concentration of bacteria gradually decreased, showing a trend similar to heavy rain, but remaining higher than in heavy rain.
Rain is known to influence the density and composition of bacteria in the air. It can affect the wet deposition of bacteria when they interact with airborne particles that are washed out of the atmosphere indirectly (Smets et al., 2016). It can be inferred that a greater amount of rain has a higher ability to wash away bacteria, resulting in a lower concentration of bacterial bioaerosols and a longer recovery period.
In this study, the concentration of bacterial bioaerosols was lower at 1 h after a heavy rain compared to both moderate and light rains, indicating the ability to remove bacteria, specifically bioaerosol particles, from the atmosphere when the rainfall amount is greater. This finding aligns with the conclusion of Zhen et al. (2017) who investigated bacterial densities after light and heavy rains and suggested that heavy rain strongly washes away suspended particles, while light rain alters the velocity of aerosol through particle deposition.
Heavy and prolonged rains provide a significant amount of water to the soil, increasing its moisture and waterlogging. Consequently, it takes longer for the ground to dry out compared to light and moderate rains, considering other factors such as solar irradiance. In our study, rainwater samples were collected during rains that occurred from 3 to 5 pm, with no high solar irradiance during that period. The moist ground hinders terrestrial microorganisms from returning to the atmosphere via convection currents (Smets et al., 2016). This hypothesis explains the difference in the trend of changing bacterial concentration between heavy rain and light–moderate rains. The bacterial concentration in the air measured from 3 to 8 h after the rain was mostly lower or equal compared to the concentration after moderate rain.
Effect of meteorological factors on the concentration of bacterial bioaerosols after the rain
To evaluate the influence of meteorological factors on the aerosol bacterial concentration after the rain, the study analyzed the variation tendency of bacterial concentration after the rain and considered the variation tendency of temperature, relative humidity, and CO2 concentration. The concentration of aerosolized bacteria after the rain generally varied similarly between rains. Specifically, it gradually increased after the rain and decreased when the peak was reached. For the temperature, a similar change was also found, the temperature peaks almost coincided with the bacterial concentrations (Fig. 2a). In contrast, relative humidity showed a distinct trend with bacterial concentration, as relative humidity increased and bacterial concentration decreased (Fig. 2b). CO2 concentrations had small fluctuation in the October and November rains, but in the September rains, CO2 concentrations changed similarly to bacteria concentrations (Fig. 2c).
The correlation between the average bacterial concentration in the three types of rain and the average temperature, relative humidity, and CO2 concentration during the 24 h after rainfall was investigated to have an insight view of the impact of meteorological factors on aerosol bacteria concentration. Figure 3 shows the variation in bacteria concentration, while Table 1 presents the results. The correlation values are mentioned in the “Meteorological conditions during sampling” section and are shown in Supplemental Figure S1.
The average concentration of airborne bacteria before rainfall and in rainwater were 271.59 ± 7.30 CFU/m3 and 274.05 ± 10.64 CFU/m3, respectively. Within 24 h after the rain, the average concentration of airborne bacteria ranged from 111.89 ± 17.98 to 264.30 ± 38.99 CFU/m3. The lowest concentration was observed 1 h after the rain event (111.89 ± 17.98 CFU/m3), and stability was recorded after 2 to 6 h. From 7 to 12 h after the rain, there was an increasing trend, with the bacterial concentration reaching its maximum, close to the pre-rainfall value (259.13 ± 56.61 CFU/m3). Between 12 and 24 h, the bacterial concentration gradually decreased and started to stabilize.
Humidity contributes to the deposition of particles in the atmosphere since suspended particles absorb ambient moisture, increasing their weight and size (Li et al., 2015). These deposited particles can adhere to other surfaces, such as leaves and buildings (Smets et al., 2016). The correlation between relative humidity and bacterial concentration was strongly negative (r = − 0.79). Rain increased the post-rainfall humidity, hindering microorganisms from returning to the atmosphere. The intensity and duration of the rain determine the level of the post-rainfall humidity. In this study, the relative humidity after rains (including light, moderate, and heavy rains) generally ranged between 70 and 90%. The concentration of airborne bacteria after the rain under the influence of light and moderate rains showed a similar trend, increasing gradually from 3 h after the rain and reaching its maximum after 12 h. Less rainfall caused the ground to dry out more quickly, allowing bacteria to return to the atmosphere at a faster rate. As a result, the concentration of microorganisms after light and moderate rains was consistently higher than that after heavy rain.
The relationship between average bacterial concentration after the rain and temperature revealed a strong positive correlation (r = 0.85). The temperature variation matched the variation in bacterial concentration. Rainfall significantly reduced the concentration of bacteria in the first 3 h after the rain due to leaching effects. Additionally, the low atmospheric temperature resulting from reduced solar heating maintained high humidity, which prevented bacteria from returning to the atmosphere. From 3 to 12 h after the rain, the temperature tended to increase, exceeding 33 °C for light rains. High temperatures above 24 °C can reduce the survival rate of microorganisms in the air (Tang, 2009). A finding on bacterial concentrations showed that this value was low at high summer temperatures but had a positive correlation with spring temperature (Zhen et al., 2017). Our study documented a gradual increase in bacterial concentration with temperature and showed that bacterial concentration was strongly positively correlated with temperature, consistent with the results in spring. It is worth mentioning that the negative impact of temperature on the survival of aerosol bacteria was also influenced by both solar radiation and temperature. Some studies showed that aerosol bacteria was influenced by the disinfecting action of intense solar radiation (Tong & Lighthart, 1997) or a high ozone concentration (Sharma & Hudson, 2008). In addition, Kang et al. (2015) investigated the effect of UV irradiation on bacterial bioaerosol concentration and found that increasing UV intensity reduced bacterial bioaerosol concentration, especially on rainy days when UV intensity was lower after the rain. In our study, the rain events typically occurred in the afternoon (3–5 pm), so the period before 14 h would be considered as nighttime (5 pm–5 am). The nighttime temperature in Ho Chi Minh City, Vietnam, was high, ranging from 30 to 32 °C, due to the tropical climate. Furthermore, the hypothesis of adaptablility related to high-temperature conditions of bacteria in cultured microbial communities (ability to adapt to different temperature conditions of tropical and temperate climates) could explain the differences observed.
From 14 to 24 h after the rain, the general trend showed a decreasing concentration of bacteria in the three types of rain, including heavy, moderate, and light rains (with heavy rain having a slightly lower value). That time period coincided with the appearance of sunlight (6 am–4 pm), during which UV radiation and temperature became unfavorable conditions for bacterial existence. In Vietnam, the sun typically begins to appear between 6 and 7 am, and the temperature and UV radiation increase year by year due to ozone depletion (with an average temperature of 29.33 °C in the sampling area). Additionally, this period marks the beginning of human activities. Vehicle emissions and heavy traffic contribute to a significant increase in airborne particles. The sampling location, near the main road at the gate of the University of Natural Sciences, is bustling with trading activities and the flow of people (students) from early morning. The emission of other biological particles and pollutants (such as SO2, NO2, CO, etc.) into the air creates competition for microorganisms. According to Gandolfi et al. (2015) and Dong et al. (2016), air pollutants can influence bacterial growth or their survival in the atmosphere. However, CO2 concentration in our study showed a positive correlation with bacterial concentration (r = 0.70). Although other air pollutants were not tested in this study, they could also contribute to competition with airborne bacteria, as suggested by the aforementioned hypothesis.
Diversity of microbial communities after rain
To study changes in airborne microbial communities before and after rainfall, we examined the microbial community structure in samples collected prior to the rain, after the rain, and in rainwater. Analysis of the bacterial community structure before rain revealed three identified phyla: Actinobacteria, Firmicutes, and Proteobacteria (Fig. 4a). Firmicutes accounted for the largest proportion (98.16%) among the three phyla, while Proteobacteria had the lowest proportion (0.37%). The predominance of Firmicutes was attributed to the order Bacillales, representing 98.16% and consisting of three families: Bacillaceae (98.157%), Paenibacillaceae (0.001%), and Staphylococcaceae (0.002%). At the genus level, it could be seen that the genus Bacillus was almost representative of the cultured bacterial community of the family Bacillaceae, appearing at the highest rate both before and after the rain (Fig. 4b). Actinobacteria, another phylum, contributed through the orders Micrococcales (0.67%), Corynebacteriales (0.60%), and Streptomycetales (0.04%). Finally, the orders within the phylum Proteobacteria included Pseudomonadales (0.35%), Burkholderiales (0.01%), Caulobacteriales (0.01%), and Enterobacterales (0.001%).
Following rainfall, Firmicutes remained the dominant phylum in the atmospheric bacterial community, with a high percentage of 99.67%. At the family level, there was a difference in the contribution rate within Firmicutes, with Bacillaceae decreasing to 94.63% and an increase observed in Paenibacillaceae and Staphylococcaceae (Fig. 4a). Additionally, the order Lactobacillales appeared in the post-rain samples, accounting for 0.003%. Notably, Streptomycetales (part of Actinobacteria) and Caulobacterales (part of Proteobacteria) were not detected in the post-rain air samples.
Rainwater samples exhibited a significantly different bacterial community structure. Proteobacteria was the most abundant phylum, accounting for 86.44%, followed by Firmicutes with 13.47%, and Actinobacteria with the lowest rate of 0.09%. Within the Proteobacteria phylum, Burkholderiales contributed the highest proportion (86.42%), with Sphingomonadales accounting for the remaining 0.02%. Figure 4b shows the highest percentage of genera Burkholderia–Caballeronia–Paraburkholderia, along with very low percentages of genera Ralstonia and Comamonas. Firmicutes, represented solely by Bacillales, consisted of Bacillaceae (8.15%) and Paenibacillaceae (5.32%), while Micrococcales (belonging to Actinobacteria) had a rate of 0.09%.
In the study, we found specific phyla of airborne bacteria, namely Firmicutes, Actinobacteria, and Proteobacteria. This aligns with previous studies conducted in Asia (Du et al., 2018; Gou et al., 2016; Xu et al., 2019). Among them, the proportions of the three dominant phyla fluctuated differently depending on many factors. Research in a central city of China recorded a higher proportion of Firmicutes than Actinobacteria (Gou et al., 2016), while some other studies showed the opposite (Du et al., 2018; Xu et al., 2019). The composition of the airborne microbial community was mentioned to change significantly in different geographical regions depending on the environmental conditions (Xiong et al., 2012). In our research, Firmicutes accounted for the highest proportion, followed by Actinobacteria, demonstrating differences in microbial communities based on geographical origin. In addition, our results show that the genus Bacillus was a typical genus of Firmicutes. The genus Bacillus is characterized by aerobic endospore-forming bacteria. Heat-resistant endospores are their most important characteristic, along with resistance to radiation, antiseptic effects, UV rays, and hygroscopicity, making Bacillus one of nature’s great survivors (Vos et al., 2009). The research by Jang et al. (2018) evaluated the influence of rain on the aerosol microbial community and showed that after rainfall, there was an increase in the abundance of Firmicutes and Actinobacteria (Jang et al., 2018), with a decrease in Firmicutes and an increase in Actinobacteria. However, our study yielded different results. We observed an increase in the Firmicutes phylum after the rain, primarily due to the higher proportion of Paenibacillaceae and Staphylococcaceae, along with a small presence of Lactobacillaceae. One possible explanation for the prevalence of Paenibacillaceae after the rain is the release of spores into the air (Bowers et al., 2012, 2013). This family belongs to the order Bacillales, which includes spore-forming bacteria (Zeigler, 2013). Warmer temperatures after rains enhance the hygroscopicity of these bacteria on surfaces, leading to spore aerosolization (Jang et al., 2018). On the other hand, Staphylococcaceae bacteria are mostly spherical and do not form spores. Many species in this order are toxic to humans and animals (Lory, 2014). Staphylococcus aureus, in particular, is commonly found in ready-to-eat street food and is a frequent food-borne pathogen (Eromo et al., 2016; Ghosh et al., 2007). In locations such as Vietnam, where street food is abundant near school gates, the presence of Staphylococcaceae bacteria in the air after rain could be attributed to aerosolization from soil or other surfaces. The occurrence of bacteria from the Lactobacillaceae family was scarce and could be explained by droplets or aerosolization from contact surfaces, potentially existing only in air samples for the first few hours. The difference in bacterial composition before and after the rain indicates the leaching of bacteria from the atmosphere with rainfall. Within the scope of the current study, bioinformatics tools have not been thoroughly applied to detect bacterial virulence genes in rainwater samples, and some biochemical testing methods to determine the pathogenicity of species have not been implemented. In addition, the culture method used in the study limited the ability to detect culturable bacteria, so several specific pathogens related to weather were not detected. Therefore, some discussions performed were speculative based on the characteristics of the sampling area. However, these preliminary findings could be the basis for further analysis of airborne bacterial composition of interest after rainfall by extracting DNA directly from environmental samples and dissecting their gene contents (virulence factors, AMR genes) with the support of bioinformatics.
Rainwater samples revealed distinct bacterial community structures. The proportion of phyla from highest to lowest were Proteobacteria, Firmicutes, and Actinobacteria. The Proteobacteria was detected at a very low rate in aerosol samples but dominated in rainwater samples. Especially, Burkholderiales—belonging to Betaproteobacteria was almost represented in all rainwater samples. Research by Kaushik et al. (2014) about bacteria composition in rainwater samples and rainwater reservoirs in Singapore also showed that Betaproteobacteria was most present in rainwater. The variation in the microbial composition between rainwater and the atmosphere could be due to differences in bacterial composition in the upper atmosphere above the sampling site, where Proteobacteria was most dominant and was carried by raindrops. Additionally, the relationship between spores and the "wet deposition" process in some spore-forming species requires further exploration.
Conclusion
Rain and other meteorological conditions like relative humidity and temperature significantly influence the concentration of bacterial bioaerosols before and after rain events. Rain contributes to wet deposition, resulting in similar bacteria concentration in rainwater samples compared to before the rain and a significant decrease in density approximately 2 h after the rain in all three types of rainfall assessed. Bacteria concentration recovers after approximately 9 h for heavy rain and 12 h for light and moderate rains. However, bacteria’s ability to return to the atmosphere after heavy rain is consistently lower compared to the other two rainfall types, reaching only 94.6% of pre-rain levels after nine hours. Relative humidity plays a role in this difference, as heavier rain makes the surface wetter, increases humidity, and hinders bacteria from emitting into the atmosphere through convection currents. This demonstrates an inverse relationship between bacterial concentration and relative humidity. On the other hand, temperature and solar radiation decrease air humidity and create conditions for bacteria to disperse into the atmosphere, showing a positive correlation with bacterial concentration. The concentration of CO2 also shows a positive correlation with bacterial concentration and requires further study along with other contaminants.
The composition of the microbial community in the air at the sampling site in Ho Chi Minh City indicated the presence of specific airborne microbial phyla in Southeast Asia, albeit with different proportions. Firmicutes account for the highest proportion (98.16%), while Proteobacteria have the lowest rate (0.16%). After the rain, the contribution level of these three phyla remains unchanged, but the proportions differ. Firmicutes increased, while the other two phyla decreased. This change is primarily due to the increased contribution of Paenibacillales and Staphylococcales, along with a small percentage of Lactobacillales, indicating a connection between aerosolization with the spore-forming bacteria group (Paenibacillales), as well as the bacterial source. Further studies need to consider extracting DNA directly from environmental samples and investigating bacterial virulence genes using bioinformatics tools to obtain representative results of the aerosol microbial community in Ho Chi Minh City, Vietnam.
Data availability
All the required data are available in the manuscript.
References
AccuWeather. (2023). AccuWeather. Retrieved April 15, 2023, from https://www.accuweather.com/
AQI. (2023). Vietnam air quality index (AQI): Real-time air pollution level. Retrieved April 20, 2023, from https://www.aqi.in/dashboard/vietnam
Arancibia, F., Ewig, S., Mensa, J., Gonzalez, J., Niederman, M. S., Torres, A., & Bauer, T. T. (2002). Community-acquired pneumonia due to gram-negative bacteria and Pseudomonas aeruginosa: Incidence, risk, and prognosis. Archives of Internal Medicine, 162(16), 1849–1858.
Bolger, A. M., Lohse, M., & Usadel, B. (2014). Trimmomatic: A flexible trimmer for Illumina sequence data. Bioinformatics (oxford, England), 30(15), 2114–2120. https://doi.org/10.1093/bioinformatics/btu170
Bolyen, E., Rideout, J. R., Dillon, M. R., Bokulich, N. A., Abnet, C. C., Al-Ghalith, G. A., Alexander, H., Alm, E. J., Arumugam, M., Asnicar, F., Bai, Y., Bisanz, J. E., Bittinger, K., Brejnrod, A., Brislawn, C. J., Brown, C. T., Callahan, B. J., Caraballo-Rodríguez, A. M., Chase, J., & Caporaso, J. G. (2019). Reproducible, interactive, scalable and extensible microbiome data science using QIIME 2. Nature biotechnology, 37(8), 8. https://doi.org/10.1038/s41587-019-0209-9
Bowers, R. M., McCubbin, I. B., Hallar, A. G., & Fierer, N. (2012). Seasonal variability in airborne bacterial communities at a high-elevation site. Atmospheric Environment, 50, 41–49. https://doi.org/10.1016/j.atmosenv.2012.01.005
Bowers, R. M., Clements, N., Emerson, J. B., Wiedinmyer, C., Hannigan, M. P., & Fierer, N. (2013). Seasonal variability in bacterial and fungal diversity of the near-surface atmosphere. Environmental Science & Technology, 47(21), 12097–12106. https://doi.org/10.1021/es402970s
Callahan, B. J., McMurdie, P. J., Rosen, M. J., Han, A. W., Johnson, A. J. A., & Holmes, S. P. (2016). DADA2: High-resolution sample inference from Illumina amplicon data. Nature Methods, 13(7), 7. https://doi.org/10.1038/nmeth.3869
CCKP. (2021). Vietnam—Climatology. World Bank Climate Change Knowledge Portal. Retrieved April 20, 2023, from https://climateknowledgeportal.worldbank.org/
Dong, L., Qi, J., Shao, C., Zhong, X., Gao, D., Cao, W., Gao, J., Bai, R., Long, G., & Chu, C. (2016). Concentration and size distribution of total airborne microbes in hazy and foggy weather. Science of the Total Environment, 541, 1011–1018. https://doi.org/10.1016/j.scitotenv.2015.10.001
Du, P., Du, R., Ren, W., Lu, Z., & Fu, P. (2018). Seasonal variation characteristic of inhalable microbial communities in PM2.5 in Beijing city, China. The Science of the Total Environment, 610–611, 308–315. https://doi.org/10.1016/j.scitotenv.2017.07.097
Eromo, T., Tassew, H., Daka, D., & Kibru, G. (2016). Bacteriological quality of street foods and antimicrobial resistance of isolates in Hawassa Ethiopia. Ethiopian Journal of Health Sciences, 26(6), 533–542. https://doi.org/10.4314/ejhs.v26i6.5
Fang, Z., Ouyang, Z., Hu, L., Wang, X., Zheng, H., & Lin, X. (2005). Culturable airborne fungi in outdoor environments in Beijing China. Science of the Total Environment, 350(1–3), 47–58. https://doi.org/10.1016/j.scitotenv.2005.01.032
FAO. (1991). Manual of food quality control. 12. Quality assurance in the food control microbiological laboratory. FAO food and nutrition paper, 14(12), 1–154.
Gandolfi, I., Bertolini, V., Bestetti, G., Ambrosini, R., Innocente, E., Rampazzo, G., Papacchini, M., & Franztti, A. (2015). Spatio-temporal variability of airborne bacterial communities and their correlation with particulate matter chemical composition across two urban areas. Applied Microbiology and Biotechnology, 99, 4867–4877.
Georgakopoulos, D. G., Després, V., Frohlich-Nowoisky, J., Psenner, R., Ariya, P. A., Pósfai, M., Ahern, H. E., Moffett, B. F., & Hill, T. C. J. (2009). Microbiology and atmospheric processes: Biological, physical and chemical characterization of aerosol particles. Biogeosciences, 6(4), 721–737. https://doi.org/10.5194/bg-6-721-2009
Ghosh, M., Wahi, S., Kumar, M., & Ganguli, A. (2007). Prevalence of enterotoxigenic Staphylococcus aureus and Shigella spp. in some raw street vended Indian foods. International Journal of Environmental Health Research, 17(2), 151–156. https://doi.org/10.1080/09603120701219204
Gou, H., Lu, J., Li, S., Tong, Y., Xie, C., & Zheng, X. (2016). Assessment of microbial communities in PM1 and PM10 of Urumqi during winter. Environmental pollution (Barking, Essex: 1987), 214, 202–210. https://doi.org/10.1016/j.envpol.2016.03.073
He, S., Goodkin, N., Jackisch, D., Ong, M. R., & Samanta, D. (2018). Continuous real-time analysis of the isotopic composition of precipitation during tropical rain events: Insights into tropical convection. Hydrological Processes, 32(1), 1531–1545. https://doi.org/10.1002/hyp.11520
Hu, W., Murata, K., Horikawa, Y., Naganuma, A., & Zhang, D. (2017). Bacterial community composition in rainwater associated with synoptic weather in an area downwind of the Asian continent. Science of the Total Environment, 601–602, 1775–1784. https://doi.org/10.1016/j.scitotenv.2017.06.052
Jang, G. I., Hwang, C. Y., & Cho, B. C. (2018). Effects of heavy rainfall on the composition of airborne bacterial communities. Frontiers of Environmental Science & Engineering, 12(2), 12. https://doi.org/10.1007/s11783-018-1008-0
Joung, Y. S., Ge, Z., & Buie, C. R. (2017). Bioaerosol generation by raindrops on soil. Nature Communications, 8(1), 14668. https://doi.org/10.1038/ncomms14668
Kang, S. M., Heo, K. J., & Lee, B. U. (2015). Why does rain increase the concentrations of environmental bioaerosols during monsoon? Aerosol and air quality research, 2320–2324. https://doi.org/10.4209/aaqr.2014.12.0328
Kaushik, R., Balasubramanian, R., & Dunstan, H. (2014). Microbial quality and phylogenetic diversity of fresh rainwater and tropical freshwater reservoir. PLoS ONE, 9(6), e100737.
Kulshrestha, U., Reddy, L., Satyanarayana, J., & Kulshrestha, M. J. (2009). Real-time wet scavenging of major chemical constituents of aerosols and role of rain intensity in Indian region. Atmospheric Environment, 43, 5123–5127.
Li, Y., Fu, H., Wang, W., Liu, J., Meng, Q., & Wang, W. (2015). Characteristics of bacterial and fungal aerosols during the autumn haze days in Xi’an, China. Atmospheric Environment, 122, 439–447. https://doi.org/10.1016/j.atmosenv.2015.09.070
Lonon, M. K. (1998). Bioaerosol sampling (indoor air) Culturable organisms: bacteria, fungi, thermophilic actinomycetes Method 0800. NIOSH Manual of analytical methods (NMAM), 1.
Lory, S. (2014). The family Staphylococcaceae. In E. Rosenberg, E. F. DeLong, S. Lory, E. Stackebrandt, & F. Thompson (Eds.), The prokaryotes: Firmicutes and Tenericutes (pp. 363–366). Springer, Berlin, Heidelberg. https://doi.org/10.1007/978-3-642-30120-9_350
Malakootian, M., & Gharghani, M. A. (2016). Investigation of type and density of bio-aerosols in air samples from educational hospital wards of Kerman city, 2014. Environmental Health Engineering and Management Journal, 3(4), 197–202.
Martin, M. (2011). Cutadapt removes adapter sequences from high-throughput sequencing reads. EMBnet Journal, 17(1), 1.
Peter, H., Hörtnagl, P., Reche, I., & Sommaruga, R. (2014). Bacterial diversity and composition during rain events with and without Saharan dust influence reaching a high mountain lake in the Alps. Environmental Microbiology Reports, 6, 618–624.
Polymenakou, P. N. (2012). Atmosphere: A source of pathogenic or beneficial microbes? Atmosphere, 3(1), 87–102. https://doi.org/10.3390/atmos3010087
Quast, C., Pruesse, E., Yilmaz, P., Gerken, J., Schweer, T., Yarza, P., Peplies, J., & Glöckner, F. O. (2013). The SILVA ribosomal RNA gene database project: Improved data processing and web-based tools. Nucleic acids research, 41, D590–D596. https://doi.org/10.1093/nar/gks1219
Seinfeld, J. H., & Pandis, S. N. (2006). Atmospheric chemistry and physics: From air pollution to climate change. Chapter 20: Wet deposition (2nd ed.). A Wiley-Interscience Publication.
Sharma, M., & Hudson, J. B. (2008). Ozone gas is an effective and practical antibacterial agent. American Journal of Infection Control, 36(8), 559–563. https://doi.org/10.1016/j.ajic.2007.10.021
Smets, W., Moretti, S., Denys, S., & Lebeer, S. (2016). Airborne bacteria in the atmosphere: Presence, purpose, and potential. Atmospheric Environment, 139, 214–221. https://doi.org/10.1016/j.atmosenv.2016.05.038
Stetzenbach, L. D. (2009). Airborne infectious microorganisms. Encyclopedia of microbiology, 175–182. https://doi.org/10.1016/B978-012373944-5.00177-2
Tang, J. W. (2009). The effect of environmental parameters on the survival of airborne infectious agents. Journal of the Royal Society Interface, 6(Suppl 6), 737–746. https://doi.org/10.1098/rsif.2009.0227.focus
Tong, Y. Y., & Lighthart, B. (1997). Solar radiation has a lethal effect on natural populations of culturable outdoor atmospheric bacteria. Atmospheric Environment, 31, 897–900.
Vos, P., Garrity, G. M., Jones D., Krieg, N. R., Ludwig, W., Rainey, F. A., Schleifer, K-H., & Whitman, W. B. (Eds.). (2009). Bergey’s manual of systematic bacteriology—The Firmicutes (2nd ed., Vol. 3). Pub by Springer. Retrieved December 3, 2023, from https://www.springer.com/series/4157
Wang, W., Ma, Y., Ma, X., Wu, F., Ma, X., An, L., & Feng, H. (2010). Seasonal variations of airborne bacteria in the Mogao Grottoes, Dunhuang. China. International Biodeterioration & Biodegradation, 64(4), 309–315. https://doi.org/10.1016/j.ibiod.2010.03.004
WHO. (2023). Air pollution in Viet Nam. World Health Organization. Retrieved April 20, 2023, from https://www.who.int/vietnam/health-topics/airpollution
Wiśniewska, K. A., Śliwińska-Wilczewska, S., & Lewandowska, A. U. (2022). Airborne microalgal and cyanobacterial diversity and composition during rain events in the southern Baltic Sea region. Scientific Reports, 12(1), 2029. https://doi.org/10.1038/s41598-022-06107-9
World Meteorological Organization. (2018). Guide to instruments and methods of observation (p. 548). World Meteorological Organization: Geneva, Switzerland.
Xiong, J., Liu, Y., Lin, X., Zhang, H., Zeng, J., Hou, J., Yang, Y., Yao, T., Knight, R., & Chu, H. (2012). Geographic distance and pH drive bacterial distribution in alkaline lake sediments across Tibetan Plateau. Environmental Microbiology, 14(9), 2457–2466. https://doi.org/10.1111/j.1462-2920.2012.02799.x
Xu, C., Wei, M., Chen, J., Zhu, C., Li, J., Xu, X., Wang, W., Zhang, Q., Ding, A., Kan, H., Zhao, Z., & Mellouki, A. (2019). Profile of inhalable bacteria in PM2.5 at Mt. Tai, China: Abundance, community, and influence of air mass trajectories. Ecotoxicology and Environmental Safety, 168, 110–119. https://doi.org/10.1016/j.ecoenv.2018.10.071
Zeigler, D. R. (2013). The Family Paenibacillaceae (p. 24). The Bacillus Genetic Stock Center.
Zhen, Q., Deng, Y., Wang, Y., Wang, X., Zhang, H., Sun, X., & Ouyang, Z. (2017). Meteorological factors had more impact on airborne bacterial communities than air pollutants. Science of the Total Environment, 601–602, 703–712. https://doi.org/10.1016/j.scitotenv.2017.05.049
Acknowledgements
The authors thank the University of Science, Vietnam National University Ho Chi Minh City. We gratefully thank the members of the Air and Water Pollution—Public Health—Climate Change research group of the Faculty of Environment, VNUHCM-University of Science for the sampling instruments. We gratefully thank Prof. Sheng-Hsiang (Carlo) Wang from National Central University, Taiwan for supporting us with the Aerobox device.
Funding
This research is funded by the University of Science, VNU-HCM under grant number T2022-69.
Author information
Authors and Affiliations
Contributions
Dang Diep Yen Nga and Vuong Hong Nhung designed and performed experiments, collected data, conducted data analysis, and wrote the manuscript. Nguyen Tri Nhan edited the manuscript. To Thi Hien helped in the preparation of the first draft of the manuscript.
Corresponding author
Ethics declarations
Ethics approval
All authors have read, understood, and have complied as applicable with the statement on “Ethical responsibilities of Authors” as found in the Instructions for authors.
Consent to participate
Not applicable.
Consent for publication
All the authors have given consent for the publication of the study.
Competing interests
The authors declare no competing interests.
Additional information
Publisher's Note
Springer Nature remains neutral with regard to jurisdictional claims in published maps and institutional affiliations.
Supplementary Information
Below is the link to the electronic supplementary material.
Rights and permissions
Springer Nature or its licensor (e.g. a society or other partner) holds exclusive rights to this article under a publishing agreement with the author(s) or other rightsholder(s); author self-archiving of the accepted manuscript version of this article is solely governed by the terms of such publishing agreement and applicable law.
About this article
Cite this article
Nga, D.D.Y., Nhung, V.H., Nhan, N.T. et al. Study on the concentration, composition, and recovery rate of bacterial bioaerosols after rainfall in Ho Chi Minh City. Environ Monit Assess 196, 295 (2024). https://doi.org/10.1007/s10661-024-12442-3
Received:
Accepted:
Published:
DOI: https://doi.org/10.1007/s10661-024-12442-3