Abstract
Periphyton is an important component of stream bioassessment, yet methods for quantifying periphyton biomass can differ substantially. A case study within the Arkansas Ozarks is presented to demonstrate the potential for linking chlorophyll-a (chl-a) and ash-free dry mass (AFDM) data sets amassed using two frequently used periphyton sampling protocols. Method A involved collecting periphyton from a known area on the top surface of variably sized rocks gathered from relatively swift-velocity riffles without discerning canopy cover. Method B involved collecting periphyton from the entire top surface of cobbles systematically gathered from riffle-run habitat where canopy cover was intentionally avoided. Chl-a and AFDM measurements were not different between methods (p = 0.123 and p = 0.550, respectively), and there was no interaction between method and time in the repeated measures structure of the study. However, significantly different seasonal distinctions were observed for chl-a and AFDM from all streams when data from the methods were combined (p < 0.001 and p = 0.012, respectively), with greater mean biomass in the cooler sampling months. Seasonal trends were likely the indirect results of varying temperatures. Although the size and range of this study were small, results suggest data sets collected using different methods may effectively be used together with some minor considerations due to potential confounding factors. This study provides motivation for the continued investigation of combining data sets derived from multiple methods of data collection, which could be useful in stream bioassessment and particularly important for the development of regional stream nutrient criteria for the southern Ozarks.
Similar content being viewed by others
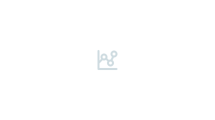
Explore related subjects
Discover the latest articles, news and stories from top researchers in related subjects.Avoid common mistakes on your manuscript.
Introduction
Nutrient enrichment remains a significant challenge in water quality management of streams, rivers, and lakes (Carpenter et al. 1998; Sharpley et al. 1994; Dodds et al. 2013). Indeed, a survey of US streams found 42% of assessed stream lengths to be in relatively poor condition, with nitrogen (N) and phosphorus (P) being two of the top four causes of impairment (Paulsen et al. 2008). The United States Environmental Protection Agency (USEPA) has requested states and tribes use empirically generated numeric nutrient criteria to assess accelerated eutrophication of streams, lakes, and estuaries (USEPA 1998). Even though the USEPA charged states and tribes with developing these criteria by 2003, as of December 2015, Hawaii is the only state that the USEPA recognizes as having both N and P criteria for all waterbody types (USEPA 2015). Specifically pertaining to streams, five states have partial N and P criteria (Arizona, California, Florida, Montana, and Nevada), three states have partial P criteria (New Mexico, Oklahoma, and Vermont), four states have statewide P criteria (Wisconsin, New Jersey, Minnesota, and Hawaii), and only Hawaii has statewide N criteria (USEPA 2015). Lack of data availability and issues related to the spatial scale of the studies, and proper data collection methods, have contributed to delays in criteria development (Evans-White et al. 2013).
One way the USEPA recommends states and tribes develop nutrient criteria for streams is through the use of predictive relationships that may be identified using bioassessment (USEPA 2000). Predictive relationships, also known as stressor-response analyses, rely on a stressor variable (e.g., N and P concentrations) and a response variable (e.g., fish, macroinvertebrate, and/or microbial assemblages) for determining quantitative relationships that characterize conditions causing impairment of particular designated uses (USEPA 2010). Periphyton is a complex assemblage of algae, cyanobacteria, heterotrophic microorganisms, and detritus that are attached to solid submersed substrates in aquatic ecosystems (Stevenson et al. 1996). Periphyton is of particular interest in stream bioassessment for developing nutrient water quality criteria because this microbial community often contains the dominant autotrophs in streams, and periphyton primary production should be directly influenced by stream water nutrient availability (Dodds et al. 2002). Periphyton biomass (e.g., mean and maximum chlorophyll-a (chl-a) and ash-free dry mass (AFDM)) have been used extensively in the literature as response variables to N and/or P as stressor variables (Evans-White et al. 2013). However, confounding factors, such as canopy cover, grazing, watershed land use, and stream flow, can influence nutrient-periphyton relationships and must also be taken into consideration during bioassessment (Biggs 2000; Rosemond et al. 2000; Wickham et al. 2005).
Multiple methods have been used throughout the USA for collecting periphyton biomass in the field (Aloi 1990; Stevenson and Bahls 1999; Lowe and LaLiberte 2007). The most common differences between methods include the following: (1) whether artificial or natural substrates are used, (2) how the substrates are selected, (3) the stream habitat (riffle, run, glide, pool, margin) from which periphyton is collected, and (4) the size of the periphyton removal area. Unfortunately, no consensus exists about which method, if any, is the most appropriate to use. Because a variety of methods is commonly used, the comparison of different studies may be complicated by unequal biases among methods. A comparative study of periphyton collection methods could benefit stream bioassessment and nutrient criteria development by clarifying the appropriateness of combining measurements derived from different sampling protocols.
Two stressor-response studies using different data collection methods in the southern Ozarks of Oklahoma and Arkansas were conducted between 2013 and 2016 and highlighted three of the four previously mentioned differences among common methods. The first study was conducted by the Arkansas Department of Environmental Quality (ADEQ) to develop nutrient criteria for Arkansas’ Extraordinary Resource Waters of Arkansas’ Ozark Highlands and Boston Mountains. ADEQ used a modified version of the USGS National Water Quality Assessment method (NAWQA; Moulton et al. 2002) for sampling periphyton, referred to hereafter as Method A. This method has also been adopted by other state agencies, such as the New Mexico Environment Department (NMED 2014). In this method, periphyton is collected from a known area using a delimiter from haphazardly selected rocks. The second study, overseen by the Arkansas-Oklahoma Scenic Rivers Joint Study (SRJS) Committee, focused on the Oklahoma-designed scenic rivers located in northeastern Oklahoma and northwestern Arkansas. The Arkansas-Oklahoma SRJS used a modified version of the USEPA periphyton sampling method, which collects periphyton from the entire top surface of systematically selected rocks (Barbour et al. 1999; King 2014) and is referred to hereafter as Method B. The goal of both studies was to develop stressor-response relationships between total P (TP) in stream water and periphyton biomass. We were interested in determining if the different methods used in these two studies would yield similar results so the data could potentially be combined into a regional stressor-response in the future (Smith et al. 2003; Smucker et al. 2013). Thus, the objective of this study was to compare the two periphyton sampling methodologies to determine if and how results varied with known confounding factors. We hypothesized that periphyton biomass collected with Method A would be systematically less than periphyton biomass collected with Method B at the same time/location because of potential bias toward more rapid current velocity and use of a smaller sampling area.
Materials and methods
Study area
The Ozarks, a level II Omernik ecoregion located in the USA, is composed of two level III ecoregions, the Boston Mountains to the south and the Ozark Highlands to the north (Omernick 1987). The dominant geology of the Ozark Highlands is limestone and dolomite, while the Boston Mountains is mainly composed of sandstone, shale, and siltstone (The Nature Conservancy 2003). Oak (Quercus), hickory (Carya), and pine (Pinus) forests dominate the Ozarks. Mean temperatures range from 8.7 to 22 °C, and mean precipitation is 115 cm annually (U.S. Climate Data 2016).
Seven southern Ozark streams were designated study sites due to similarities in geology, drainage area, land use, and proximity to the two Ozark stressor-response studies (Table 1; Fig. 1). Drainage areas ranged from 130 km2 at Cave Creek to 368 km2 at the Little Buffalo River (USGS 2015). Land use was predominantly forested in all watersheds, ranging from 75.4 to 94.3% at Bear Creek and White River, respectively (Table 1). Sampled stream sections were primarily gravel and cobble dominated, which is a common stream condition throughout the Ozarks (Panfil and Jacobson 2001). All sample streams originate in the Boston Mountains and drain north into the Ozark Highlands (Fig. 1). Big Creek, Cave Creek, Little Buffalo River, and Bear Creek are also tributaries of the Buffalo River, which is designated as an Extraordinary Resource Water by the state of Arkansas. The study streams were centrally located between the Arkansas-Oklahoma SRJS field sites and the ADEQ Extraordinary Resource Waters field sites (Fig. 1). We originally sought study streams along an anthropogenic nutrient concentration gradient, but preliminary analysis of stream water samples revealed similar ranges in total N (TN) and TP across all sample sites throughout the study (Table 1). Thus, the relationships between algal biomass and nutrient concentrations were not evaluated in this study. Instead, the primary goal was to investigate potential periphyton biomass variability across methods.
Map of study stream locations (yellow triangles), Arkansas-Oklahoma (AR/OK) Scenic Rivers Joint Study range (green squares), and the Arkansas Department of Environmental Quality (ADEQ) Extraordinary Resource Waters study range (red circles). U.S. level III ecoregion GIS layer provided by USEPA. State boundaries GIS layer provided by the ESRI, TomTom, U.S. Department of Commerce, and U.S. Census Bureau
In-stream sampling and data collection
The two periphyton sampling methods are described in detail below. Briefly, Method A involved collecting periphyton from small delimited areas on various sized cobble substrate from streams (Aloi 1990). Alternatively, Method B involved collecting periphyton from the entire top surface of systematically selected cobble substrate and estimating the surface area from which periphyton was removed (Barbour et al. 1999; King 2014). Other major differences between the two methods include the type of stream habitat sampled, cobble selection method, cobble size, number of cobbles collected, and canopy cover preference (Table 2). Methods A and B were used to collect periphyton from each study stream over a 1-year period, and specific details of each sampling method are provided below. Periphyton sampling commenced in mid-August 2014 with consecutive sampling occurring roughly every 3 months thereafter (i.e., November 2014, February 2015, June 2015, and August 2015) to capture seasonal changes. Flow, habitat characteristic data, and water chemistry data were collected during each sampling event. We used both methods to collect periphyton on the same day in each stream. All seven streams were sampled within a 2-week time period during each season, unless unforeseen weather-related circumstances prevented sampling due to safety issues or periphyton scouring events. When major scouring events were observed, sampling was postponed 2 weeks to allow periphyton re-establishment. We measured flow in a single location during each quarterly sampling event using the mid-point method with a Marsh-McBirney Inc. Flo-mate (model #2000, Frederick, MD). Water samples were collected from each site monthly for nutrient analysis.
Method A
We selected riffles by visual observation of relatively turbulent water velocity and retrievable substrate and avoided areas where periphyton had been scoured or vegetation covered the stream bed. No preference to canopy cover was given when making riffle selections, but streams with open canopy were generally selected because of a preference existing in method B. Two riffles were selected at each stream, with the riffle at the lowest downstream location sampled for periphyton first. An attempt was made to sample upstream of bridges; however, this was often unavoidable due to study site characteristics. The head and toe of each riffle were identified using the best professional judgment. Five evenly spaced rocks representative of the stream bed environment were collected in an approximate straight line from the right to the left bank across the head and toe of each riffle. Rocks were placed in clean white containers as they were collected in the stream and covered with stream water to prevent periphyton drying out. We avoided selecting rocks that had obviously been scoured or were embedded in the stream bed. A total of 20 primarily cobble-sized rocks (10 rocks/riffle) was collected per stream for this method. Size was not a main consideration when making rock selections; however, as mentioned previously, the stream reaches were generally dominated by gravel to cobble-sized rocks.
A rubber gasket (i.e., delimiter) with a 6.35-cm outer diameter and a 2.54-cm inner diameter was placed on the top surface of each rock (Fig. 2a). The periphyton inside the inner area of the gasket was removed (Fig. 2b) and placed into a clean white container using a metal Scoopula and/or a small brush with plastic bristles using as little stream water as possible for rinsing. Care was taken to minimize detachment of periphyton outside of the designated removal area. Periphyton removed from the 10 rocks collected from each riffle was combined into a single sample so that a periphyton sample was obtained for each of the two riffles. Periphyton samples from each riffle were stored separately in labeled 1-L acid-washed dark bottles and placed on ice until transported to the laboratory at the University of Arkansas, Fayetteville, AR.
Habitat characteristic data were recorded for each riffle. We recorded cross-sectional area at 10 evenly spaced points along the total riffle length by measuring the stream depth at mid-channel and near the left and right banks. We estimated mean seasonal velocity for Method A habitat in each stream using each cross-sectional area measurement with the single stream flow measurement from the reach. Percent closed canopy cover was measured using a concave densiometer at the lower, middle, and upper sections of each riffle.
Method B
We selected riffle-run habitat at each site with relatively open canopy cover and cobble substrate. We avoided areas in streams that exhibited obvious periphyton scouring, any rooted vegetation, and depths greater than 30 cm. The number of riffles selected for sampling varied depending on the availability of ideal sampling habitat. For example, sometimes one long riffle-run was selected, but other times three separate riffles were selected and sequentially sampled from downstream to upstream. As with Method A, attempts were made to reduce the number of samples collected directly downstream of bridges; however, this was often unavoidable. Three transects were placed along the wetted width of the selected riffle-run habitat, with the first transect placed farthest downstream. Transects were positioned a minimum distance apart, which was no less than the length of the longest transect. Starting with the most downstream transect and progressing to the consecutive upstream transects, five large washers with flagging tape (referred to as “markers”) were systematically placed in the stream equal distances apart along each transect. One cobble-sized rock (10–20 cm2) was collected within a 1-m2 area of each marker that best represented periphyton growth in that respective part of the stream. Embedded rocks were avoided. Selected rocks were placed in clean white containers organized by transect number, and containers were filled with stream water to avoid periphyton drying out. Rocks were photographed with identifying information to visually document periphyton biomass.
Periphyton were removed from the entire top surface of each rock using a small brush with wire bristles and a minimal volume of stream water. The resulting slurry was compiled for all transects into 1-L acid-washed dark bottles and placed on ice until transported to the laboratory at the University of Arkansas, Fayetteville, AR. Aluminum foil was trimmed to match the area scraped on each rock. A linear regression of area by weight of the aluminum foil was used to convert foil weight to the area of each rock scraped.
Percent canopy cover and velocity were recorded at the center marker of each transect using a concave densiometer and Marsh-McBirney Inc. Flo-mate, respectively. Depth and qualitative measurements, such as dominant substrate, sedimentation on a scale of 1 to 20, percent embeddedness, and percent filamentous cover, were recorded at each marker. We estimated mean seasonal velocity for Method B habitat in each stream using measured mean wetted width and mean depth from the riffle habitats and the stream flow measured as described previously.
Laboratory analyses
Periphyton samples from both sampling methods were individually homogenized with a handheld blender, and total slurry volumes were recorded for each sample per stream within 48 h of sample collection. Each slurry was separately mixed on a magnetic stirring plate, and subsamples of the mixed slurries were individually filtered onto 25-mm Whatman glass-fiber filters (GF/F) for analysis of periphyton biomass as chl-a. Subsamples were also filtered onto preweighed 47-mm Whatman GF/F that had been combusted at 450 °C for AFDM analysis. All samples were then frozen at −20 °C for analysis on subsequent dates. A Turner Designs Trilogy Fluorometer (model #7200-000, Sunnyvale, CA) was used to analyze samples for periphtyon chl-a following the acetone extraction method (APHA 2005; #10200 H). Ash-free dry mass was estimated following sample drying and ashing at 450 °C (APHA 2005; #10300 C). Subsample chl-a and AFDM results and the calculated area of periphyton removal were used to compute biomass collected per square meter for slurries amassed using each method. Water samples were analyzed for TN and TP on a Skalar San++ Continuous Flow Analyzer (Skalar Inc., The Netherlands) (APHA 2005; #4500-N C and #4500-P F) at the Arkansas Water Resource Center’s Certified Laboratory, Fayetteville, AR, following persulfate digestion (APHA 2005; #4500-P J).
Data analysis and statistics
Samples collected using Method A yielded two periphyton biomass measurements (one per riffle) for each stream reach sampled on each date, while method B yielded just one periphyton biomass measurement for each reach on each date. Preliminary data analysis using Welch two sample t tests revealed no significant difference in periphyton biomass (chl-a or AFDM) between upstream and downstream riffles sampled using Method A (p = 0.638, t = 0.474, and df = 63.9 and p = 0.559, t = 0.587, and df = 55.8, respectively). Thus, the average periphyton chl-a and AFDM from both riffles were used to represent results from Method A.
To compare results from the two methods, the Mixed Procedure in SAS (Version 9.4; Cary, NC) was used to run a repeated measures analysis of variance (ANOVA) with a random block design for both periphyton AFDM and chl-a. Because there was little variation in TP among streams, we had no a priori hypothesis about potential differences in periphyton biomass among streams. We considered stream to be a random effect for this reason and to account for potential autocorrelation. Method was the main effect, and time was treated as a repeated measure. Potential method and time interactions were taken into consideration using an unstructured covariance matrix. The model assumed that all correlations and variances could be different. Two chl-a data points (War Eagle Creek in August 2014 and White River in August 2015) and three AFDM data points (War Eagle Creek in June 2015, White River in June 2015, and August 2015) were excluded in the final data analysis because insufficient material was collected onto filters, which caused measurement errors near the level of detection for the instrumentation.
In addition to the statistical comparison of methods, we also computed paired chl-a, AFDM, velocity, and canopy cover differences between methods by subtracting Method B results from Method A results. This was done to graphically examine the degree of difference between methods across seasons and explore any possible relationships between numerical differences in periphyton biomass and numerical differences in variables that we expected to potentially confound the direct nutrient control of periphyton biomass in streams. We also calculated the absolute value of each biomass difference and the 5th and 25th percentiles of all chl-a and AFDM measurements collected throughout the study from all streams and methods. From these calculations, we compared the magnitude of difference in periphyton biomass between methods to the actual measured periphyton biomass.
Results
Method and time comparisons
As shown in Table 3, chl-a and AFDM did not differ between Method A and Method B (p = 0.123 and p = 0.550, respectively) across our study sites. Additionally, neither chl-a nor AFDM differed between methods over time (p = 0.270 and p = 0.200, respectively). However, chl-a and AFDM differed through time, regardless of sampling method (p < 0.001 and p = 0.012, respectively).
The seasonal variability in periphyton biomass created a range in chl-a and AFDM that demonstrated the proportionality of Methods A and B (Fig. 3a, b). Interestingly, the chl-a results from Method A were often greater than the chl-a results from Method B (i.e., many data <1:1 line in Fig. 3a). The trend in numerical differences between methods was primarily driven by observations in November, February, and June (Fig. 4a, b). However, there were no numerical differences in AFDM (Fig. 4c, d), and the repeated measures ANOVA indicated that the numerical differences in chl-a between methods were not statistically significant (Table 3).
Seasonal and site specific a chorophyll-a and b ash-free dry mass results collected using Method A and Method B. Dashed line represents 1:1 relationship. Colors represent seasons: August 2014 (red), November 2014 (green), February 2015 (blue), June 2015 (pink), and August 2015 (black). Symbols represent streams: Bear Creek (black square), Big Creek (black circle), Cave Creek (black triangle), Kings River (black diamond), Little Buffalo River (operator), War Eagle Creek (plus sign), and White River (eight-spoked asterisk)
a Seasonal influence on chlorophyll-a (chl-a) differences between both methods for all streams and b seasonal influence by site on chl-a differences between both methods. c Seasonal influence on ash-free dry mass (AFDM) differences between both methods for all streams and d seasonal influence by site on AFDM differences between both methods. Differences were calculated by subtracting Method B biomass results from Method A biomass results. Symbols represent streams: Bear Creek (white square), Big Creek (white circle), Cave Creek (white triangle), Kings River (black diamond), Little Buffalo River (operator), War Eagle Creek (plus sign), and White River (eight-spoked asterisk). The blue diamonds are the means of each response variable, and the solid middle lines are the medians of each response variable
Because periphyton biomass did not differ between methods, results from both methods were combined into a one-way ANOVA to compare specific differences among seasons (i.e., August as summer, November as fall, February as winter, and June as spring) using least squares means differences. Mean chl-a collected from all streams using both methods was greatest in November and February (116.7 and 108.8 mg/m2, respectively) and least in June and August (60.0 and 70.6 mg/m2, respectively) (Fig. 5a). Mean AFDM collected from all streams using both methods was also greatest in November and February (69.8 and 58.4 g/m2, respectively) and least in June and August (40.0 and 38.7 g/m2, respectively) (Fig. 5b). However, AFDM measured in February did not differ from that measured in June and August 2015. February AFDM was greater than AFDM measured in August 2014 by 23.6 g/m2.
Seasonal influence on (a) all chlorophyll-a (chl-a) and (b) all ash-free dry mass (AFDM) collected from all streams using both methods throughout the study. The blue diamonds are the means of each response variable, and the solid middle lines are the medians of each response variable. Significant differences are designated by letters (a, b, and c)
Relationships with confounding factors
Mean discharge throughout the study was the lowest in September 2014 (0.20 m3/s) and peaked in May 2015 (23.3 m3/s) before the June sampling event (Table 4). Mean velocity differences between methods across all sites, calculated by subtracting Method B velocity values from Method A velocity values, ranged from −1.4 m/s in June to 0.5 m/s in November (Fig. 6a). The most variability in numerical velocity differences between methods was in June, but numerical velocity differences were minimal throughout the study (Fig. 6a). A paired t test showed there was no difference in velocity of the habitats sampled for either method (p = 0.511, t = −0.665, and df = 32). We also observed similarities in percent closed canopy cover between habitats sampled using both methods in each stream throughout the study (Fig. 6b). Specifically, mean percent closed canopy cover from each stream across all seasons ranged from 17.5% in February to 22.9% in August 2015 and 16.6% in November to 26.1% in August 2014 for habitats sampled using Method A and Method B, respectively. Mean differences in percent closed canopy cover between habitats sampled using both methods across all streams, calculated by subtracting Method B canopy cover values from Method A canopy cover values, ranged from −1.5% in November to 4.5% in August 2014 (Fig. 6b). A paired t test showed no difference in the canopy cover of the habitats sampled for either method (p = 0.588, t = 0.547, and df = 33). Thus, mean percent canopy cover of habitats sampled using both data collection methods did not explain small numerical differences between methods either in chl-a or AFDM data collected from each stream across seasons.
Seasonal influence on a differences in velocity between habitats sampled in all streams using both methods and b percent closed canopy cover differences between methods. Differences were calculated by subtracting Method B results from Method A results. The blue diamonds are the means of each response variable, and the solid middle lines are the medians of each response variable
Discussion
Methods that quantify periphyton biomass are extremely important in stream bioassessment and in developing numeric water quality standards for nutrients. However, existing methods for quantifying periphyton are diverse and may not address common confounding factors equally. Results of this study indicated the whole-surface and the delimiter-reduced periphyton removal methods used by the Arkansas-Oklahoma SRJS and ADEQ, respectively, yielded similar results at the level II ecoregion scale and across seasons. The lack of difference between methods was notable because there was significant seasonal variability in periphyton biomass, regardless of which method used.
Although numerical variations in measurements were observed between methods, the magnitude of these differences was small compared to the magnitude of variability in periphyton biomass over the course of the study. For example, the numeric differences in chl-a in November and February (~50 mg/m2; Fig. 4a, b) sampling was similar in magnitude to the total periphyton biomass measured in other months (Fig. 5a). Otherwise, variations in chl-a and AFDM values between methods were relatively small when considering overall chl-a and AFDM measurements. Thus, the variation between methods was not statistically significant, and the numerical variation among methods was usually small compared with the numerical (and statistically significant) variation in periphyton biomass among seasons. Although we observed periphyton biomass in excess of 100 mg/m2, which Welch et al. (1988) identify as the threshold for nuisance conditions, we never observed excessively long (i.e., > 10 cm) filamentous algal biomass in any of the streams sampled in the study. Because Method A uses a small delimiter size, we expect that this method would have significantly underestimated filamentous algae like Cladophora sp. if it were growing in meter- or multi-meter-long filaments (Dodds and Gudder 1992).
Seasonal influences on periphyton biomass
Temporally changing biological processes are known to influence periphyton biomass accrual in streams (e.g., temperature, grazing, flow, light, and nutrient availability) and have been well documented in the literature (Ceola et al. 2013; Lange et al. 2011; Winkelmann et al. 2014). Periphyton biomass was greatest during the cool seasons when water temperatures were less than or equal to 10 °C. Periphyton growth rates typically increase with water temperature up to approximately 30 °C (DeNicola 1996). Increased sloughing of attached algae has been observed with temperature increases between 23.5 and 30 °C and may have contributed to the decreasing trend in periphyton biomass during the warmer months of June and August (Dodds and Gudder 1992; Whitton 1970). However, the effect of temperature was probably indirect because temperatures greater than or equal to 30 °C were minimal during our study. Thus, greater periphyton biomass in November and February was perhaps caused by decreased grazing pressures or variable light availability due to changing deciduous tree canopies (Hillebrand 2009; Quinn et al. 1997). However, streams sampled in this study had minimal canopy cover, which was not directly related to variation in periphyton biomass among streams (Figs. 5 and 6).
Riparian vegetation is known to influence the amount of photosynthetically active radiation (PAR) available to periphyton communities, which can control biomass accumulation (Lowe et al. 1986; Hill 1996). The Ozarks are dominated by deciduous forest (The Nature Conservancy 2003), which can translate to seasonally dynamic light conditions in streams (Halvorson et al. 2016). Although operational guidelines for collecting canopy cover measurements were less strict for Method A, canopy cover results happened to be similar between Method A and Method B throughout the entire study. This result, along with the fact that we targeted open-canopy environments with Method B, suggests canopy cover was not the primary factor influencing seasonal differences in periphyton biomass in this study. Nutrient concentrations remained relatively similar for each stream throughout the duration of the study, but the availability of reactive nutrients was not measured (Dodds et al. 2002). Flow conditions also varied seasonally (Table 4), and increased flow has been shown to increase periphyton biomass (Biggs et al. 2005) up until the point where scouring can decrease periphyton biomass. However, any quantitative threshold in flow that could induce scouring in our study streams remains unknown and excessive large flow events did not occur during this study. Instead, seasonal grazing pressure appears to be a logical explanation for the variation observed in periphyton biomass in this study.
Grazer and algal community structures often display seasonal changes, and edibility of algae can vary throughout the year (Vanni and Temte 1990). For example, algal community composition changes can be coupled with changes in grazer or scraper community composition (DeNicola et al. 1990). In this study, increased filamentous algae biomass was visually observed in streams during the cooler months, which may have been caused by, or resulted in, shifts in macroinvertebrate functional feeding groups due to preferential feeding (Dodds and Gudder 1992; Hawkins and Sedell 1981). In contrast to this study, Rosemond (1994) did not detect seasonal trends in periphyton accrual and attributed the observation to large quantities of grazing snails. Thus, the dynamic nature of grazing as a potential confounding factor must be explicitly considered in stream bioassessment methods that involve periphyton biomass quantitation.
Seasonal water temperatures may indirectly influence periphyton biomass accumulation in streams through variation in grazing pressure by fishes. Two crayfish species, Cambarus chasmodactylus and Orconectes cristavarius, and three benthic fish species, northern hogsuckers (Hypentelium nigricans), white suckers (Catastomus commersoni), and central stonerollers (Campostoma anomalum), exhibited reduced feeding activities during winter when the mean temperature was less than 6 °C in a temperate North Carolina river (Fortino 2006). Increased feeding activities of the same crayfish species were previously documented in the same river during August when mean temperatures were relatively warmer (Helms and Creed 2005). Dewey (1981) reported seasonal changes in small fish abundances in a southern Ozark stream, implying grazing pressures also varied throughout the year. Specifically, the central stoneroller (C. anomalum) is one of the most abundant fish species in Ozark streams, making them an important control on periphyton biomass due to their strong grazing influences (Gelwick and Matthews 1992; Robison and Buchanan 1988; Matthews et al. 1987). Temperature-mediated impacts on trophic interactions observed in a boreal stream mesocosm experiment showed increased predatory fish (Salvelinus malma) feeding activity at 12 °C led to decreased caddisfly (Glossosoma spp.) grazing and increased periphyton biomass accrual (Kishi et al. 2005). Decreased feeding activity of the predacious fish at 21 °C allowed caddisfly grazing to reduce periphyton biomass (Kishi et al. 2005). Evans-White et al. (2003) observed preferential seasonal feeding on algae by two crayfish known to occur in Arkansas, Orconectes nais and O. neglectus, with greater grazing occurring in spring compared to that in summer or fall. Results from these studies support the idea that grazing pressures may have been reduced during November and February in all streams during our study when the mean temperature was 8 °C.
Study implications
The large-scale implication of this study is that periphyton biomass collected from cobbles using the whole-surface periphyton removal method versus the delimiter-reduced periphyton removal method resulted in no measureable differences. Variations in velocity and light between the methods may have minimally influenced comparability of periphyton biomass. However, these variations were not statistically significant and were small relative to the seasonal changes in periphyton biomass. More specifically, these results suggest managers should use discretion, but may combine data from these different methods in order to generate sufficiently large databases to support nutrient and periphyton biomass relationships if streams do not have excessively long filamentous algae growth. For example, the Arkansas-Oklahoma SRJS data and the ADEQ Extraordinary Resource Waters data may be linked together in regional stressor-response analyses for the ultimate goal of deriving regional nutrient criteria for streams and rivers of the southern Ozarks. Although there was no method by season interaction observed in this study, the data exhibited heteroscedasticity (i.e., the magnitude of difference between methods and the measured periphyton biomass was positively correlated). Thus, fair caution may be to only combine data sets collected in seasons with similar temperature ranges so as to avoid any seasonally dynamic confounding factors.
The limited spatial scale and small number of sample sites in this study provide a brief glimpse into the possibilities of combining data sets collected with Method A and Method B. Canopy cover in this study was similar between habitats sampled using both methods. Thus, more extreme variations in canopy cover may have greater implications for methodological differences. Additionally, grazing macroinvertebrates and fish are known to influence standing stocks of periphyton in aquatic habitats, especially where large numbers of grazers reside (Hillebrand 2008; Hillebrand et al. 2008; Taylor et al. 2012). We were unable to quantitatively sample for grazers in this study due to time constraints. However, anecdotal evidence suggests grazing pressure may strongly control periphyton biomass in Ozark streams (Power et al. 1988). Different types of algae also are better suited for certain habitats and variable temperatures and can produce varying amounts of biomass (Biggs and Hickey 1994; Lange et al. 2011). Taxonomic identification of periphyton communities was not conducted for samples in this study. Quantitatively identifying the impacts of differences between habitats sampled, primarily, variations in periphyton community composition, canopy cover, and grazing influences are important considerations for future method comparison studies.
In an ideal situation, scientists and natural resource managers would agree to use one method of data collection and hold true to that decision. In reality, existing data sets that used different methods of data collection already exist and each investigator has reasons why one method may be better to use over another method (Aloi 1990). Consensus about which method to use is unlikely to happen in the foreseeable future, so using the best available information to justify combining data sets is pertinent because combining data sets increases the availability of data for use in bioassessment and nutrient criteria development. Although these findings suggest there was no significant difference between AFDM and chl-a results collected using the whole-surface and the delimiter-reduced periphyton removal methods, future studies with larger sample sizes may yield other results, and caution should be applied when combining data from different studies.
Conclusion
The goal of this study was to assess the comparability of two common periphyton sampling protocols for the purpose of advancing the development of water quality criteria for nutrients. Results demonstrated that there were no discernable differences in data based on the method of data collection. Sharp differences in periphyton biomass were observed between the warmer (i.e., August and June) and cooler months (i.e., November and February) of this study, with the cooler months yielding more variable results. Although we did not measure grazing directly, we observed less activity by grazing fish in cooler months, which is supported by literature on their temperature preferences, suggesting that grazing may strongly limit periphyton biomass accumulation in warm months. These results suggest that estimates of chl-a and AFDM from each method are comparable. However, it is very important to note that we never observed long filamentous algal growth by species such as Cladophora. In streams where these long filamentous species dominate, the delimeter method may be particularly limited in application and the whole-rock method is likely preferable.
References
Aloi, J. (1990). A critical review of recent freshwater periphyton field methods. Canadian Journal of Fisheries and Aquatic Sciences, 47, 656–670.
APHA (American Public Health Association) (2005). Standard methods for the examination of water and wastewater, 21st edn. In A. D. Easton, L. S. Clesceri, E. W. Rice, A. E. Greenburg (Eds). Washington D.C.: American Public Health Association, American Water Works, Water Environment Federation.
Barbour, M. T., Gerritsen, J., Snyder, B. D., & Stribling, J. B. (1999). Rapid bioassessment protocols for use in streams and wadeable rivers: periphyton, benthic macroinvertebrates, and fish. EPA 841/B-99/022. Washington, D.C.: Office of Water, US Environmental Protection Agency.
Biggs, B. J. F. (2000). Eutrophication of streams and rivers: dissolved nutrient-chlorophyll relationships for benthic algae. Journal of North American Benthological Society, 19(1), 17–31.
Biggs, B. J. F., & Hickey, C. W. (1994). Periphyton responses to a hydraulic gradient in a regulated river in New Zealand. Freshwater Biology, 32, 49–59.
Biggs, B. J. F., Nikora, V. I., & Snelder, T. H. (2005). Linking scales of flow variability to lotic ecosystem structure and function. River Research and Applications, 21, 283–298.
Carpenter, S. R., Caraco, N. F., Correll, D. L., Howarth, R. W., Sharpley, A. N., & Smith, V. H. (1998). Nonpoint pollution of surface waters with phosphorus and nitrogen. Ecological Applications, 8(3), 559–568.
Ceola, S., Hödl, I., Adlboller, M., Singer, G., Bertuzzo, E., Mari, L., et al. (2013). Hydrologic variability affects invertebrate grazing on phototrophic biofilms in stream microcosms. PloS One, 8(4), 1–11. doi:10.1371/journal.pone.0060629.
DeNicola, D. M. (1996). Periphyton responses to temperature at different ecological levels. In R. J. Stevenson, M. L. Bothwell, & R. L. Lowe (Eds.), Algal ecology: freshwater benthic ecosystems (pp. 149–181). San Diego: Academic Press.
DeNicola, D., McIntire, C. D., Lamberti, G. A., Gregory, S. V., & Ashkenas, L. R. (1990). Temporal patterns of grazer-periphyton interactions in laboratory streams. Freshwater Biology, 23(3), 475–489.
Dewey, M. R. (1981). Seasonal abundance, movement, and diversity of fishes in an Ozark stream. Arkansas Academy of Science Proceedings, 35, 33–39.
Dodds, W. K., & Gudder, D. A. (1992). The ecology of Cladophora. Journal of Phycology, 415–427.
Dodds, W. K., Smith, V. H., & Lohman, K. (2002). Nitrogen and phosphorus relationships to benthic algal biomass in temperate streams. Canadian Journal of Fisheries and Aquatic Sciences, 59(5), 865–874. doi:10.1139/f02-063.
Dodds, W. K., Perkin, J. S., & Gerken, J. E. (2013). Human impact on freshwater ecosystem services: a global perspective. Environmental Science & Technology, 47, 9061–9068. doi:10.1021/es4021052.
Evans-White, M. A., Dodds, W. K., & Whiles, M. R. (2003). Ecosystem significance of crayfishes and stonerollers in a prairie stream: functional differences between co-occurring omnivores. Journal of the North American Benthological Society, 22(3), 423–441. doi:10.2307/1468272.
Evans-White, M. A., Haggard, B. E., & Scott, J. T. (2013). A review of stream nutrient criteria development in the United States. Journal of Environmental Quality, 42(4), 1002–1014. doi:10.2134/jeq2012.0491.
Fortino, K. (2006). Effect of season on the impact of ecosystem engineers in the New River, NC. Hydrobiologia, 559(1), 463–466. doi:10.1007/s10750-005-5325-5.
Gelwick, F. P., & Matthews, W. J. (1992). Effects of an algivorous minnow on temperate stream ecosystem properties. Ecological Society of America, 73(5), 1630–1645.
Halvorson, H. M., Scott, E. E., Entrekin, S. A., Evans-White, M. A., & Scott, J. T. (2016). Light and dissolved phosphorus interactively affect microbial metabolism, stoichiometry, and decomposition of leaf litter. Freshwater Biology, 61, 1006–1019.
Hawkins, C., & Sedell, J. (1981). Longitudinal and seasonal changes in functional organization of macroinvertebrate communities in four Oregon streams. Ecology, 62(2), 387–397. doi:10.2307/1936713.
Helms, B. S., & Creed, R. P. (2005). The effects of 2 coexisting crayfish on an Appalachian river community. Journal of the North American Benthological Society, 24(1), 113–122.
Hill, W. R. (1996). Factors affecting benthic algae. In R. J. Stevenson, M. L. Bothwell, & R. L. Lowe (Eds.), Algal ecology: freshwater benthic ecosystems (pp. 31–56). San Diego: Academic Press.
Hillebrand, H. (2008). Grazing regulates the spatial variability of periphyton biomass. Ecological Society of America, 89(1), 165–173.
Hillebrand, H. (2009). Meta-analysis of grazer control of periphyton biomass across aquatic ecosystems. Journal of Phycology, 45(4), 798–806. doi:10.1111/j.1529-8817.2009.00702.x.
Hillebrand, H., Frost, P., & Liess, A. (2008). Ecological stoichiometry of indirect grazer effects on periphyton nutrient content. Oecologia, 155, 619–630. doi:10.1007/s00442-007-0930-9.
King, R. S. (2014). Oklahoma scenic rivers joint phosphorus study work plan. https://www.ok.gov/conservation/documents/Baylor%20University%20Contract%20and%20Workplan.pdf. Accessed 11 Jan 2016.
Kishi, D., Murakami, M., Nakano, S., & Maekawa, K. (2005). Water temperature determines strength of top-down control in a stream food web. Freshwater Biology, 50(8), 1315–1322. doi:10.1111/j.1365-2427.2005.01404.x.
Lange, K., Liess, A., Piggott, J. J., Townsend, C. R., & Matthaei, C. D. (2011). Light, nutrients and grazing interact to determine stream diatom community composition and functional group structure. Freshwater Biology, 56(2), 264–278. doi:10.1111/j.1365-2427.2010.02492.x.
Lowe, R. L., & LaLiberte, G. D. (2007). Benthic stream algae: distribution and structure. In F. R. Hauer & G. A. Lamberti (Eds.), Methods in stream ecology. San Diego: Academic Press.
Lowe, R. L., Golladay, S. W., & Webster, J. R. (1986). Periphyton response to nutrient manipulation in streams draining clearcut and forested watersheds. Journal of the North American Benthological Society, 5(3), 221–229.
Matthews, W. J., Stewart, A. J., & Power, M. E. (1987). Grazing fishes as components of North American stream ecosystems: effects of Campostoma anomalum. Community and Evolutionary Ecology of North American Stream Fishes, 128–135.
Moulton II, S. R., Kennen, J. G., Goldstein, R. M., & Hambrook, J. A. (2002). Revised protocols for sampling algal, invertebrate, and fish communities as part of the national water-quality assessment program. Open-File Report 02–150. Reston: U.S. Department of the Interior, U.S. Geological Survey.
NMED (New Mexico Environment Department). (2014). Periphyton sampling. SOP 11.2. Surface Water Quality Bureau. https://www.env.nm.gov/swqb/SOP/11.2SOP-PeriphytonSampling2014.pdf. Accessed 3 March 2016.
Omernick, J. M. (1987). Ecoregions of the conterminous United States. Annals of the Association of American Geographers, 77, 118–125.
Panfil, M. S., & Jacobson, R. B. (2001). Relations among geology, physiography, land use, and stream habitat conditions in the Buffalo and Current River systems, Missouri and Arkansas. ISSN 1081-292X. US Department of the Interior, US Geological Survey, Biological Resources Division, Columbia Environmental Research Center, Columbia, Missouri.
Paulsen, S. G., Mayio, A., Peck, D. V., Stoddard, J. L., Tarquinio, E., Holdsworth, S. M., et al. (2008). Condition of stream ecosystems in the US: an overview of the first national assessment. Journal of the North American Benthological Society, 27(4), 812–821. doi:10.1899/08-098.1.
Power, M. E., Stewart, A. J., & Matthews, W. J. (1988). Grazer control of algae in an Ozark mountain stream: effects of short-term exclusion. Ecology, 69(6), 1894–1898. doi:10.2307/1941166.
Quinn, J. M., Cooper, A. B., Stroud, M. J., & Burrell, G. P. (1997). Shade effects on stream periphyton and invertebrates: an experiment in streamside channels. New Zealand Journal of Marine and Freshwater Research, 31(5), 665–683. doi:10.1080/00288330.1997.9516797.
Robison, H. W., & Buchanan, T. M. (1988). Fishes of Arkansas. Fayetteville: University of Arkansas Press.
Rosemond, A. D. (1994). Multiple factors limit seasonal variation in periphyton in a forest stream. Journal of the North American Benthological Society, 13(3), 333–344.
Rosemond, A. D., Mulholland, P. J., & Brawley, S. H. (2000). Seasonally shifting limitation of stream periphyton: response of algal populations and assemblage biomass and productivity to variation in light, nutrients, and herbivores. Canadian Journal of Fisheries and Aquatic Sciences, 57(1), 66–75. doi:10.1139/f99-181.
Sharpley, A. N., Chapra, S. C., Wedepohl, R., Sims, J. T., Daniel, T. C., & Reddy, K. R. (1994). Managing agricultural phosphorus for protection of surface waters: issues and options. Journal of Environment Quality, 23, 437–451. doi:10.2134/jeq1994.00472425002300030006x.
Smith, R. A., Alexander, R. B., & Schwarz, G. E. (2003). Natural background concentrations of nutrients in streams and rivers of the conterminous United States. Environmental Science & Technology, 37, 3039–3047.
Smucker, N. J., Becker, M., Detenbeck, N. E., & Morrison, A. C. (2013). Using algal metrics and biomass to evaluate multiple ways of defining concentration-based nutrient criteria in streams and their ecological relevance. Ecological Indicators, 32, 51–61. doi:10.1016/j.ecolind.2013.03.018.
Stevenson, J. R., & Bahls, L. L. (1999). Periphyton protocols. In Rapid bioassessment protocols for use in streams and wadeable rivers: periphyton, benthic macroinvertebrates, and fish (pp. 1–23).
Stevenson, R. J., Bothwell, M. L., & Lowe, R. L. (1996). Algal ecology: freshwater benthic ecosystems. San Diego: Academic Press.
Taylor, J. M., Back, J. A., & King, R. S. (2012). Grazing minnows increase benthic autotrophy and enhance the response of periphyton elemental composition to experimental phosphorus additions. Freshwater Science, 31(2), 451–462. doi:10.1899/11-055.1.
The Nature Conservancy, Ozarks Ecoregional Assessment Team. (2003). Ozarks ecoregional conservation assessment. Minneapolis: The Nature Conservancy Midwestern Resource Office 48 pp. + 5 appendices.
U.S. Climate Data. (2016). Climate Ozarks-Arkansas. http://www.usclimatedata.com/climate/ozark/arkansas/united-states/usar0428. Accessed 11 Jan 2016.
USEPA (US Environmental Protection Agency). (2000). Nutrient criteria technical guidance manual rivers and streams. EPA-822-B-00-002. Washington, D.C.: US Environmental Protection Agency, Office of Science and Technology, Office of Water.
USEPA (US Environmental Protection Agency). (2010). Using stressor-response relationships to derive numeric nutrient criteria. EPA-820-S-10-001. Washington, D.C.: US Environmental Protection Agency, Office of Water.
USEPA (US Environmental Protection Agency). (2015). State development of numeric criteria for nitrogen and phosphorus pollution. US Environmental Protection Agency, Washington, D.C. http://cfpub.epa.gov/wqsits/nnc-development/. Accessed 28 Dec 2015.
USEPA (US Environmental Protection Agency). (1998). EPA national strategy for the development of regional nutrient criteria. EPA-822-R-98-002. US Environmental Protection Agency, Office of Water, Washington, D.C.
USGS (US Geological Survey). (2015). StreamStats. Version 3.0. http://water.usgs.gov/osw/streamstats/arkansas.html. Accessed 28 Dec 2015.
Vanni, M. J., & Temte, J. (1990). Seasonal patterns of grazing and nutrient limitation of phytoplankton in a eutrophic lake. Limnology and Oceanography, 35(3), 697–709. doi:10.4319/lo.1990.35.3.0697.
Welch, E. B., Jacoby, J. M., Horner, R. R., & Seeley, M. R. (1988). Nuisance biomass levels of periphytic algae in streams. Hydrobiologia, 157, 161–168.
Whitton, B. A. (1970). Biology of Cladophora in freshwaters. Water Research, 4, 457–476. doi:10.1016/0043-1354(70)90061-8.
Wickham, J. D., Riitters, K. H., Wade, T. G., & Jones, K. B. (2005). Evaluating the relative roles of ecological regions and land-cover composition for guiding establishment of nutrient criteria. Landscape Ecology, 20(7), 791–798. doi:10.1007/s10980-005-0067-3.
Winkelmann, C., Schneider, J., Mewes, D., Schmidt, S. I., Worischka, S., Hellmann, C., & Benndorf, J. (2014). Top-down and bottom-up control of periphyton by benthivorous fish and light supply in two streams. Freshwater Biology, 59, 803–818. doi:10.1111/fwb.12305.
Acknowledgements
Thank you to the Natural Resource Conservation Service and the State of Arkansas for providing the funding for this study and to Ryan King and Tate Wentz for their input on the study design. The Buffalo River National Park was gracious in allowing us to conduct some of this research within the park boundaries. Much appreciation is given to Amie West, Shannon Speir, Toryn Jones, Justin Woodruff, Sara Hallett, Delaney Hall, Nathan Sorey, Tim Glover, April Price, Harrison Smith, Kayla Sayre, Jessalyn Kohn, Matthew Rich, Byron Winston, and Deanna Mantooth for contributing many hours of field and laboratory assistance. We also thank Edward Gbur for statistical consultations.
Author information
Authors and Affiliations
Corresponding author
Rights and permissions
About this article
Cite this article
Rodman, A.R., Scott, J.T. Comparing two periphyton collection methods commonly used for stream bioassessment and the development of numeric nutrient standards. Environ Monit Assess 189, 360 (2017). https://doi.org/10.1007/s10661-017-6085-1
Received:
Accepted:
Published:
DOI: https://doi.org/10.1007/s10661-017-6085-1