Abstract
Biochars are used as amendments to improve soil quality, but their effects on edaphic organisms such as earthworms remain controversial. This study aimed to assess the effects of adding a poultry manure-derived biochar into a contaminated technosol on trace element (TE) (i.e. As, Cd, Cu, Pb, and Zn) bioavailability for two earthworm species, Aporrectodea icterica and Aporrectodea longa. Three components of the bioavailability concept were determined using a pot experiment: (1) total soil TE (potentially reactive) and TE concentrations in the soil pore water (environmental availability), (2) TE concentrations in depurated whole earthworm bodies (environmental bioavailability) and (3) ecophysiological and biochemical effects on earthworms (toxicological bioavailability). Biochar addition increased TE concentrations in the soil pore water respectively from 1.8, 2.7, 9.4, 0.7 and 959 to 6, 6.2, 19.3, 6.9, and 3003 µg L−1 for As, Cd, Cu, Pb and Zn. Biochar addition did not influence TE environmental bioavailability for earthworms, except a decreased As concentration (32.5 to 15.2 µg g−1) in A. icterica. This suggests an inter-specific variability in As homeostasis in the Aporrectodea genus. In line with this internal As decrease, the Glutathione-S-transferase (GST) activity decreased by 42% and protein and lipid contents slightly increased (14 and 25%, respectively) in A. icterica tissues. The body weight of both earthworm species decreased for the biochar-amended soil. Environmental TE availability depended on both the biochar addition and the earthworm activity in the contaminated soil, while environmental and toxicological bioavailabilities resulted from the earthworm species, the targeted TE and biochar supply to the soil.
Similar content being viewed by others
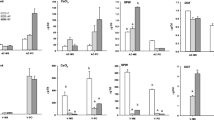
Explore related subjects
Discover the latest articles, news and stories from top researchers in related subjects.Avoid common mistakes on your manuscript.
Introduction
Anthropogenic activities result in increasing areas of degraded land worldwide (Gibbs and Salmon 2015), potentially leading to depletion in soil organic matter (Bot and Benites 2005) and to soil contamination (FAO and ITPS 2015). Trace elements (TE) can be defined as essential and non-essential metal(loid)s with common concentrations in plant shoots below 100 mg kg−1 dry weight (DW) (Adriano 2001). Anthropogenic TE excess has been identified by the European Commission as one of the eight major threats to European soils (Kidd et al. 2015). It affects soil organisms (Ponge 2013; Lüscher et al. 2014), notably earthworms (Lévêque et al. 2015; Pass et al. 2015). Earthworms represent a large proportion of soil living biomass (Yasmin and D’Souza 2010) and are pivotal in many soil processes (Bhadauria and Saxena 2010; Blouin et al. 2013). They improve soil physical conditions through the incorporation and fragmentation of organic matter (OM) and the formation of soil aggregates and porosity (Pulleman et al. 2005; Coq et al. 2007). They are also involved in nutrient cycling, thus positively influencing plant growth and health (Scheu 2003; Blouin et al. 2005). Earthworms, living in close contact with the soil, are exposed to contaminants through epidermis contact and soil ingestion. They can accumulate contaminants with potential impacts on their metabolism, survival rate and reproduction (Nahmani et al. 2007; Beaumelle et al. 2014). Earthworms are recognized as vectors of contaminants (e.g. TE) in terrestrial food webs (Scheifler et al. 2006; Fritsch et al. 2012).
In the context of restoration of degraded land (Menz et al. 2013) and particularly for soil quality issues, biochars are used as soil amendments to improve the carbon sequestration and crop yields while recycling crop residues and stabilizing soil contaminants (Park et al. 2011; Liu et al. 2013; Zhang et al. 2013a, b). Biochars are stable carbon-rich materials synthesized through pyrolysis/carbonization of plant- and animal-based biomass (Ahmad et al. 2014; Kuppusamy et al. 2016). Biochar addition in soils is an old technique originated from slash-and-burn agriculture, which emerges as a promising method to limit the TE mobility in soils and their transfer cascades to living organisms (Beesley et al. 2010; Zhang et al. 2013b). However, from short-term negative impacts to long-term null effects of biochars on earthworm feeding behavior, growth, survival rate, reproduction, population dynamics and cell integrity were reported (Weyers and Spokas 2011). Due to their reactivity towards TE, biochars may modulate their bioavailability for biological receptors such as earthworms (Beesley et al. 2011; Ahmad et al. 2014; Buss et al. 2015). Bioavailability is defined in the ISO 17402 (2008) as a dynamic process including three steps: (1) environmental availability indicates contaminant pools in the soil that can potentially be taken up by the organism; (2) environmental bioavailability designates the process of contaminant uptake by organisms; and (3) toxicological bioavailability represents the contaminant effects on these organisms (Lanno et al. 2004; Harmsen 2007). In many ecotoxicological studies dealing with TE effects on earthworms, authors compared the environmental TE availability and TE concentrations in body tissues, but few studies dealt simultaneously with the different components of bioavailability (Nahmani et al. 2007; Nirola et al. 2016). Finally, according to Beesley et al. (2011), “studies on the toxicity of biochar to earthworms […] are both scarce and contradictory”, and notably conducted in contaminated soils.
This study aimed to assess the effects of adding a poultry manure-derived biochar into a contaminated technosol on trace element bioavailability for earthworms. In a pot experiment, two species of earthworms, Aporrectodea icterica (Savigny, 1826) and Aporrectodea longa (Ude, 1885), were exposed to a As, Cd, Cu, Pb and Zn-contaminated technosol either amended or not amended with a poultry manure-derived biochar. Environmental trace element availability was quantified by measuring TE concentrations in the soil pore water. Environmental bioavailability was assessed by measuring TE concentrations in depurated whole earthworm bodies. Finally, toxicological bioavailability for earthworms was investigated by measuring their survival rate, body weight changes, energy reserves (protein, lipid and glycogen contents) and the GST (glutathione-S-transferase) enzymatic activity as an indicator of detoxification processes. Based on the available literature, we assumed that the biochar amendment would decrease the components of TE environmental and toxicological bioavailability for earthworms.
Materials and methods
Soil and biochar characteristics
The contaminated technosol (FAO World Reference Base, named hereafter the F soil) is a silty loam soil collected from the top 0–25 cm at the PHYTOSED scale 1- platform (1 ha, Fresnes-sur-Escaut, France) in February 2012 (see supplementary material S1). It has developed over dredged sediments of the Escaut River channel deposited at this dumpsite over the last century. Soil parameters and pseudo-total element concentrations (aqua regia extraction) are reported in Table 1. The potential soil ecotoxicity was assessed using the ISO 17512-1 (2008) avoidance test with Eisenia fetida (Savigny, 1826). Earthworms were more attracted (18%) to the F soil than to the ISO soil (10% sphagnum peat, 20% kaolinite clay, 70% industrial quartz sand, and 0.3 to 1.0% calcium carbonate).
The biochar was produced in a commercial pyrolysis reactor by Florentaise (Saint Mars du Désert, France). The biomass feedstock consisted of poultry manure. The pyrolysis temperature peaked at 420 °C for 180 s. This biochar was used as supplied, without prior washing to remove soluble salts. The pH of the poultry manure-derived biochar (PMB) was 10.3. Its elemental composition and carbon content concentration are shown in Table 2 while its polycyclic aromatic hydrocarbon, PAH) concentrations are reported in S2. Total Cr, Cu, Ni, and Zn concentrations in the biochar (respectively 183, 362, 75 and 1100 mg kg−1) were higher than the European Biochar Certificate V4.8 threshold values but in the same ranges as the IBI biochar standards (European Biochar 2016) (Table 2).
Experimental design
At the end of March 2014, the F soil was air-dried and sieved at 5 mm. Soil sample aliquots (2 kg DW) were placed in 4 L plastic pots to constitute four treatments: barren-unamended F soil (F soil); F soil with earthworms (F-E); biochar-amended F soil (F-B) and biochar-amended F soil with earthworms (F-BE) (n = 4 replicates per treatment). The biochar (2% air-dried soil, w/w) was carefully mixed with the soil using a vat for the F-B and F-BE treatments. Concentrations of main TE contaminants in the soil F (i.e. As, Cu, Pb and Zn) varied respectively (in mg kg−1) from 42.9, 110, 956, and 6089 to 42, 115, 937, and 5989 after a 2% biochar addition. Soils were watered with tap water (composition in S3) and maintained daily at 70% of their water holding capacity (WHC) in a greenhouse at the Centre INRA-Bordeaux Aquitaine (Villenave d’Ornon, France). During the setup, one rhizon MOM moisture sampler (Eijkelkamp, The Netherlands) was inserted with a 45° angle into each potted soil. In all treatments, 28 days after the beginning of the experiment, a 30 mL-sample of soil pore water was collected (three times 10 mL with a 12-hour interval between each sampling). This sample was kept at 4°C prior to TE analysis by ICP-AES (Varian liberty 200, Germany) and ICP-MS (Thermo X series 200, USA) (Table 3). For convenience, we referred here to element concentration in the soil pore water as soluble element concentration. Soil pH and electrical conductivity (EC) were measured twice, i.e. at the beginning and at the end of the experiment. For that, 50 mL of milli-Q water were mixed with 10 g of air-dried soil. The mixture was allowed to react for 2 h before measurements (ISO 10390 2005) (S4).
Earthworms
Juveniles of A. icterica and A. longa were collected by hand-sorting from an uncontaminated fallow in Versailles, France (48°47’ N, 2°04’ E), that had not received chemicals for more than 20 years. In previous works in this fallow, adult individuals have been identified at the species level according to the identification key of Sims and Gerard (1999). Juveniles were identified by morphological characteristics of the species and to the specific form they take in formalin in comparison with that of identified adults. They were kept under laboratory conditions, at 15 ± 2 °C, in the same uncontaminated soil for at least two weeks before the experiment. The soil moisture content was adjusted to 70% of the WHC. Both earthworm species are commonly found in French pastures and cultivated fields (Bouché 1972; Blakemore 2000), but belong to two different ecological earthworm groups. They exhibit morphological and ecological differences with respect to their burrowing behavior (Bouché 1972). Endogeic species such as A. icterica construct (sub)horizontal burrows. They can take advantage of the burrowing activities of other species such as anecic ones (e.g., A. longa) that construct vertical burrows (Jégou et al. 2001; Sheehan et al. 2007). According to these differences, we assumed contrasting behaviors of both earthworm species in terms of TE bioavailability.
Prior to use, earthworms were removed from the cultures, rinsed in tap water and weighed without voiding their gut contents to avoid stress due to fasting. To take into account their weight differences (weight of A. longa is about seven times the one of A. icterica), one individual of A. longa and seven individuals of A. icterica were randomly placed at the surface of each pot at the beginning of the experiment. Pots were closed using geotextile (nylon mesh) to prevent earthworms from escaping. After 28 days (based on ISO 11268-2 1998), living earthworms were removed from the pots and weighted without voiding their gut contents. For measuring TE concentrations in earthworms, they were individually depurated for 48 h in Petri dishes on damp filter paper in the dark at 15 ± 2 °C (Spurgeon et al. 2000; Koster et al. 2006) before being frozen at −80°C. As depuration is an additional stress, these depurated individuals were not used for biochemical measurements. Due to their few number, A. longa individuals were only used for TE measurements, whereas A. icterica individuals were split in two batches for further TE or biochemical analyses.
Measurements on earthworms
Earthworm survival rate and weight gain
The earthworm survival rate and individual weight gain were measured after 28 days of exposure. The weight gain was determined using the following equation, from Martin (1986):
where WT0 and WT28 are the weights measured at Day 0 and Day 28 after exposure respectively, without depuration.
TE concentrations in earthworm bodies
Each A. longa individual and between two and four individuals of A. icterica (depending on the survival rate in each pot) were used for measuring As, Cd, Cu, Pb and Zn concentrations in depurated earthworm bodies, after freeze-drying and wet-digestion. For each pot, the A. longa sample or the sample made of two to four A. icterica were digested with 3 mL of HNO3 68% (VWR, Analytical grade) in TFM© reactors at 180°C during 15 min in a CEM Mars Express system (Matthews, California, USA). The final volume was adjusted to 20 mL with ultra-pure water before analysis by flame atomic absorption spectrometry (SpectrAA 220, Varian). Quantification limits (mg L−1) were: Cu 0.03, Zn 0.009, Pb 0.04, and Cd 0.004.
Biochemical measurements in A. icterica
Two or three A. icterica individuals (depending on the survival rate in each pot) were used for all biochemical measurements, which were carried out in triplicate for each individual. Frozen samples were individually homogenized using an ultra-turrax (IKA T10, at 15000 rpm) in 1 mL of ice-cold phosphate buffer 100 mM at pH 7.2, 1 mM EDTA per 100 mg of earthworm. A portion of the homogenate was centrifuged 30 min at 9000 rpm and 4 °C. Glutathione-S-Transferase (GST) activities were measured in the fresh supernatant, immediately after homogenization. Energy reserves were measured on the homogenate frozen at −20 °C. Protein contents were determined in the supernatant and in the total homogenate after freezing at −20°C, using the bicinchoninic acid method and bovine serum albumin as a standard (Smith et al. 1985).
GST activity was assayed as described by Habig et al. (1974). The substrate used was 1-chloro-2,4-dinitro-benzene (CDNB). Earthworm supernatants were diluted 1:2 (v:v) with the phosphate buffer. A 4 µL aliquot of each dilute sample was mixed with the assay buffer to achieve the following final conditions: 100 mM phosphate buffer, 2 mM GSH and 1 mM CDNB in a final volume of 200 µL. The formation of reduced glutathione (GSH)-CDNB complex was monitored at 340 nm (ε = 9.6 mM−1.cm−1) for 5 min at 25 °C, after an initial 1 min incubation step. Reagent blanks (without sample) and negative controls (without GSH) were included to ensure measurement quality. The final GST activity was corrected for nonspecific reaction and was expressed as µmol of GSH-CDNB complex produced per min per milligram of protein.
Glycogen was quantified in the tissue homogenates according to Holmstrup et al. (2011) with slight modifications. 150 µL of tissue homogenate was kept in 50 µL 2 M NaOH at 80°C for 3 h and 100 µL of the extraction mixtures were incubated for 2 h at 37°C with 50 µL of 20 mg mL−1 amyloglucosidase (Aspergillus niger, Sigma-Aldrich) in 850 µL of 0.25 M acetate buffer pH 4.75. Glucose was quantified using hexokinase reagent (Sigma-Aldrich) by measuring absorbance at 340 nm. Glycogen contents were calculated relative to a glycogen standard curve (Bovine liver glycogen, Sigma-Aldrich).
Lipids were extracted following Folch et al. (1957). Tissue homogenates (500 µL) were stored at −20 °C in 1.25 mL methanol until analysis. Chloroform was added to achieve a methanol/chloroform ratio of 2:1 (v:v). After centrifugation (5 min, 1700 rpm), lipids were separated from the water-soluble material by adding 1 volume of chloroform followed by 1 volume of milliQ water (Millipore, 18 MΩ) to achieve a volume ratio of 1:1:0.3. After a second centrifugation (5 min, 1700 g), the chloroform layer was evaporated using nitrogen flux. The extraction mixtures were incubated with 5 mL of sulfuric acid in boiling water for 10 min, cooled down on ice for 5 min, and 200 µL of each sample was mixed with 3 mL of phosphoric acid-vanillin reagent freshly prepared following Knight et al. (1972). After an incubation of 15 min at 37 °C, optical density at 525 nm was read and lipid contents were calculated relative to olive oil standards (Sigma-Aldrich).
Statistical analyses
The effect of biochar addition on the trace and major element concentrations in the soil pore water was tested using two-way ANOVA(s) (Table 3). A Principal Component Analysis (PCA) was also conducted on soluble As, Cd, Cu, Pb and Zn concentrations after a 28-day exposure to the F soil, amended or not with biochar, and in presence/absence of earthworms (S5). The effects of biochar amendment on the survival rate and weight changes of both earthworm species, and As, Cd, Cu, Pb and Zn concentrations in whole-worm bodies were tested with a two-way ANOVA (Generalized Linear Model, binomial distribution for the survival rate and Linear Model, normal distribution for the other parameters) (Figs. 1 and 2, S6). A student-t-test was conducted to evaluate the impact of biochar amendment on the GST activity and the total lipid, protein and glycogen contents for A. icterica (Fig. 3, S6). One-way ANOVA(s) were used to test the effect of biochar addition on soil pH and electrical conductivity at the beginning and the end of the experiment (S4). Normality of residuals and homoscedasticity were met for these tests. Post hoc tests were also performed to assess multi-comparisons of means. Normality of residuals and homoscedasticity were met for the student-t-test, but only after a Log-Transformation of data in the specific case of total protein contents. Statistical analyses were done using the R statistical software (version 3.0.1, R Development Core Team 2011).
Aporrectodea icterica (in light grey) and Aporrectodea longa (in dark grey): a survival rate (%) and b weight changes (%) after a 28-day exposure to soils unamended (F-E soils) and amended with a poultry-manure-derived biochar (F-BE soils) (A. icterica, n = 25, A. longa n = 4). Values are means ± SD. The different letters stand for statistical significance among the treatments at the 0.05 level with a multiple pairwise comparison test
a Cd, b Cu, c Pb, d Zn and e As concentrations (μg g−1 DW) in Aporrectodea icterica (in light grey) and Aporrectodea longa (in dark grey) after a 28-days of exposure to soils unamended (F-E soil) and amended with poultry-manure derived biochar (F-BE soil). Values are means ± SD (n = 4, except for (Pb) A. longa F-BE soil n = 2 and (As) A. longa F-BE soil n = 3). The different letters stand for statistical significance between the treatments at the 0.05 level with a multiple pairwise comparison test. Dashed line indicate internal Cd/Cu/Zn concentrations in tissues of A. longa under uncontaminated conditions (Qiu et al. 2013)
a Total protein content (mg protein g earthworm−1, FW), b total glycogen content (mg g earthworm−1, FW), c total lipid content (mg g earthworm−1, FW), and d Glutathione-Stransferase (GST) activity (μmole min−1 mg protein−1) in Aporrectodea icterica after a 28-day exposure to soils unamended (F-E soil) and amended with poultry-manure-derived biochar (FBE soil, n = 12). Values are means ± SD. Stars (*) stand for statistical significance at the 0.05 level with Pairwise comparisons using t tests, adjusted with a Bonferroni correction. Dashed line indicate total glycogen and lipid concentrations and the GST activity in tissues of A. longa under uncontaminated conditions (Pelosi et al. 2016)
Results
Element concentrations in the soil and the soil pore water—environmental availability
Total soil Cd, Pb and Zn in the F soil largely surpassed both the inquiry threshold values for French soils (Baize et al. 2007) but also French guideline values for dredged-sediment management (Arrêté du 9 août 2006), i.e. 5-, 10-, and 20-fold (Table 1). Total soil As exceeded this regulatory standard by 1.4 fold, while total soil Cu only exceeded the inquiry threshold value for French soils by 3 fold, but not the French guideline values for dredged-sediment management. Soluble As, Cu and Pb concentrations in the F soil did not reflect total soil As, Cu and Pb (Table 3). Conversely, soluble Zn and Cd concentrations were higher in the F soil than in the uncontaminated one (Table 3).
The presence of earthworms increased the soluble Cd and Zn concentrations. The biochar amendment enhanced the soluble Cd, Cu, Pb and Zn concentrations (Table 3). A significant interaction between biochar and earthworms was found, underlining the modulation of environmental Al, As, Fe, Mo, Ni, and P availability by earthworms after biochar addition into the F soil. Soluble Mo and Ni concentrations peaked in the F-B soil, but decreased in the F-BE soil as compared to the F-B one. Conversely, soluble As, Fe and P concentrations were higher in the F-B soil than in the F one but they peaked in the F-BE soil. Similarly, soluble Al peaked in the F-BE soil, but it was the lowest in the F-B soil. Regarding the nutrient elements, a similar significant interaction between biochar and earthworms led to higher soluble K, Mg and Na concentrations in the F-B soil as compared to the F soil, but soluble Ca, K, Mg and Na concentrations peaked in the F-BE soil (Table 3).
Trace element concentrations in earthworm tissues—environmental bioavailability
Cadmium, Cu and Pb concentrations in earthworm bodies were in the same range for A. icterica and A. longa, i.e. on average 16 ± 4, 36 ± 10, and 29 ± 12 µg g−1 DW, respectively across the four treatments (Fig. 2a-c). Zinc concentrations in whole-worm bodies were, on average in all treatments, significantly higher in A. longa than in A. icterica (respectively, 2092 and 516 µg g−1 DW) but it was not influenced by the biochar amendment (Fig. 2d, S.4). Conversely, As concentration in whole-worm bodies was lower in A. longa than in A. icterica (Fig. 2e). Moreover, biochar amendment in the F soil significantly decreased it in comparison to the non-amended soil (from 32 to 15 µg As g−1 DW) for A. icterica. Similar trends occurred for environmental Cd and Cu bioavailability in the F-BE soil but differences were not significant for both metals (Fig. 2a, b, e; S6).
Earthworm survival rate, weight gain, energy reserves and GST activity
The survival rate for A. longa was 100% in both the F-BE and F-E soils. For A. icterica, it was 90% in the F-E soil and 82% in the F-BE soil, but the differences were not significant between species and also between treatments (Fig. 1a, S6). The weight of both earthworm species decreased after 28 days of exposure in the F soil but the highest significant weight loss was observed with biochar addition for both species (Fig. 1b, S6).
Energy reserves and GST activity were measured in A. icterica. Biochar amendment did not influence total glycogen content in A. icterica tissues. However total protein and lipid contents were respectively 15 and 26% higher in the F-BE A. icterica tissues than in the F-E one (Fig. 3a–c). Conversely, the GST activity in tissues was 41% lower for the F-BE soil (Fig. 3d).
Discussion
Environmental availability
Effects of the biochar on soil characteristics
The biochar amendment increased the soil electrical conductivity as well as the concentrations of As, Cd, Cu, Ni, Mo, Pb and Zn in the soil pore water. This was partly unexpected. As mentioned in the section 2.1, our biochar displayed total TE concentrations in line with the EBC and IBI threshold values (Table 2). Therefore, its incorporation should have led to a slight increase in total soil Al, Ca, Cr, Cu, K, Na, Ni, Mg, and P but also to a slight decrease in total As, Cd, Pb and Zn concentrations in the F-B and F-BE soils. Moreover, biochar has been claimed to immobilize metallic cations by (1) cation exchange (Lu et al. 2012), (2) both specific (surface inner-sphere complexes) and non-specific adsorption (outer-sphere complexes) (Park et al. 2011), (3) increase in the soil pH and cation retention on soil particles (Houben et al. 2013; Rees et al. 2014), and (4) TE reactions with P and Ca, leading to the formation of more stable forms of TE complexes and less TE solubility (Rajapaksha et al. 2015). Here, soil pH remained steady (around 7) in the four treatments. Changes in TE-speciation thus could not be driven by this parameter. Further spectroscopic studies (e.g. XRD, EXAFS) may evidence chemical TE speciation in the F soil, amended or not with the biochar. On another hand, biochars pyrolyzed at a temperature lower than 500°C (here 420°C) may display a high dissolved organic carbon (DOC) content (Ahmad et al. 2014) that may contribute to solubilize major or trace elements (Beesley and Dickinson 2011; Rees et al. 2014). Wagner and Kopenjohann (2014) reported increased Cu and Pb leaching correlated with increased DOC concentrations after biochar addition. Similarly, Oustrière et al. (2016) reported a 3-fold increase of both dissolved organic matter (DOM) and Cu concentrations in the soil pore water of a Cu-contaminated soil amended with the same biochar as in our study. Further investigations are required on the F soil amended or not with biochar to evaluate whether the DOC provided by our biochar was complexing with TE as organo-metal complexes, thus bringing them into solution. Moreover, in the F-B soil, total and soluble P concentrations higher than in the F soil (Tables 2 and 3) suggested possible competition between arsenates, molybdates and phosphates for chemical reactions and sorption sites, leading to highest soluble As and Mo in the F-B soil. Competition for binding sites between Mg, Ca, Na and K (provided by the biochar) and Al, Cd, Cr, Cu, Ni, Pb and Zn may have promoted the solubilization of these cations. In addition, high chloride concentration in this biochar may result in soluble chloro-complexes for Cd, Cu, Ni and Zn. Biochar amendment was also expected to stimulate the soil microbial activity (Fischer and Glaser 2012) which in turn might have complicated TE speciation and solubilization (Gregory et al. 2015).
Effects of earthworms on soil characteristics
In this study the presence of earthworms influenced soil characteristics, e.g. electrical conductivity and TE availability. Zinc and Cd were more soluble in the soil pore water in the presence of earthworms. In the F-BE soil, compared to the F-B one, such Zn and Cd solubilization by earthworms was lower in the presence of biochar. Along with the As solubilization in the F-BE soil as compared to the F-B one, these effects could be due to (i) the direct impact of earthworm activity, e.g., burrowing, passage through the earthworm gut, mucus production, and changes in DOC and (ii) an indirect impact of this activity, through the stimulation of the microbial communities (Blouin et al. 2013; Bernard et al. 2012). Our findings confirmed that earthworms are able to influence the environmental TE availability, even on a short-term basis (Sizmur and Hodson 2009; Natal-da-Luz et al. 2011), but also that biochar may have a buffered action on this influence.
The highest soluble Al, Cd, Cu, Zn, Ca, Mg, P and K concentrations occurred in the biochar-amended F-BE soil with A. icterica and A. longa (Table 3). Earthworms may have contributed to element mobilization by acting on biochar-derived DOC and DOM. Generally, earthworms such as E. fetida do not avoid biochar-amended soils compared to unamended soils (Chan et al. 2008). However, some species such as Pontoscolex corethrurus in the Amazonian terra preta soils can push aside the biochar particles to avoid ingesting them (Topoliantz and Ponge 2005). According to Beesley et al. (2011), it is so far not clear yet whether earthworm effects act by disturbance or ingestion of biochar.
Environmental bioavailability
The biochar did not influence TE bioavailability for earthworms, except for As that was lower in A. icterica tissues in the biochar-amended treatment (Fig. 2). Although the biochar addition increased the availability of some TE in soils (see section 4.1.), it may also lead to a reduced feeding activity of earthworms (Topoliantz and Ponge 2005; Gomez-Eyles et al. 2011), thus decreasing the amount of TE ingested. Gomez-Eyles et al. (2011) reported no or small differences in TE accumulation in earthworm bodies after exposure to a biochar amended-soil contaminated by PAH and TE, likely due to such reduced feeding activity. Similarly, in our study (see section 4.4), earthworms displayed a higher weight loss in the soils amended with biochar.
The phosphate fraction provided by the biochar may have contributed to increase soluble As (see above). At the same time, monovalent phosphate (H2PO4 −) may have competed with As for uptake through transporter-mediated mechanisms in cells of A. icterica. Lee and Kim (2008) reported similar differential As accumulation and toxicity in E. fetida depending on the chloride and phosphate concentrations in the soil. The interspecific variability of As accumulation that was 3-fold lower in A. longa than in A. icterica whole body remains here unclear. One hypothesis is a differential production of potential ligands such as a metallothioneins and phytochelatins between species in response to As exposure, as described by Liebeke et al. (2013) for Lumbricus rubellus at the intra-specific level. Morgan et al. (2004) previously described such intense metallothioneins immunostaining detectable in chloragogenous tissue throughout the body of L. rubellus.
For both F-BE and F-E soils (i.e., with and without biochar, respectively) Zn concentration was roughly 4 fold higher in A. longa than in A. icterica. The A. longa internal Zn concentration after 28 days of exposure to the contaminated F soil was 11 fold higher than what Qiu et al. (2013) reported in whole-worm bodies of A. longa under uncontaminated conditions. Spurgeon et al. (2000) reported an ability to regulate the internal Zn concentrations in Aporrectodea caliginosa exposed to a gradient of Zn concentration in soils. Similarly, Beaumelle et al. (2015) found stable Zn partitioning for A. caliginosa exposed to soils with total Zn varying from 40 to 1000 mg kg−1. According to these authors, such findings confirm the assumption of an active regulation of internal Zn concentration in A. caliginosa (Spurgeon and Hopkin 1999). Conversely, our results were in line with Morgan and Morgan (1998) who reported higher Zn concentrations in tissues of A. caliginosa from a contaminated soil than in A. caliginosa from an unpolluted soil. Dai et al. (2004) confirmed a significant correlation between total soil Zn and its accumulation in A. caliginosa. Internal As and Zn concentrations followed reverse trends in our study, the highest As concentrations in earthworm tissues being found for A. icterica, while internal Zn concentrations peaked in A. longa tissues. Similarly to our suggestion on internal As concentrations (see above), changes in ligand production (e.g. metallothioneins) and Zn trafficking in tissues of A. longa in response to increasing Zn exposure may explain its higher internal Zn concentrations (Kowald et al. 2016). Such findings suggest an inter-specific variation of Zn and As homeostasis in the Aporrectodea genus.
For both F-BE and F-E soils, Cd, Cu and Pb concentrations in whole-worm bodies were similar for both earthworm species (Fig. 2). Internal Cd and Cu concentrations in tissues of A. longa after a 28 days exposure to the F soils were respectively 18 and 3.5 fold higher than in whole-worm bodies of A. longa under uncontaminated conditions (Qiu et al. 2013). Internal Cd concentrations of both earthworm species were in the range determined for A. caliginosa exposed to soils with total Cd increasing up 10 mg kg−1 (Beaumelle et al. 2015). However, they were lower than the values reported in A. longa exposed up to 15 mg Cd kg−1 in soils (Morgan and Morgan 1992). Beaumelle et al. (2015) suggested that earthworms can accumulate high amounts of Cd after short term exposure to high available soil Cd concentrations (e.g. Giska et al. 2014). Internal Pb concentrations were in the upper range or above those for A. caliginosa exposed to soils with total Pb reaching up to 800 mg kg−1 (Beaumelle et al. 2015). However, our values were lower than internal Pb concentrations reported by Morgan and Morgan (1992) in tissues of A. longa exposed to a 3 820–10 110 mg Pb kg−1 gradient in soils. This confirmed the high variability of Pb internal concentration in earthworms described by Beaumelle et al. (2015).
Toxicological bioavailability
In the F-E soil, the GST activity for A. icterica was higher than in a previous study under uncontaminated conditions (Pelosi et al. 2016). This suggests that an oxidative stress occurred in A. icterica individuals exposed to the contaminated F-E soil. This GST activity decreased in worms from the F-BE soil, in line with enhanced protein and lipid contents, likely reflecting a lower oxidative stress in presence of biochar. This would match with the lower internal As concentration of A. icterica exposed to the biochar-amended F-BE soil. Even though the biochar did not affect the survival rate of the individuals, both species suffered from a higher weight loss in the biochar-amended soils than in the F soil (Fig. 1). Similarly, corn stover-biochar negatively affects the growth of A. caliginosa (Hale et al. 2013). This may be explained by an avoidance of biochar particles resulting in a reduction in their feeding activity. Moreover, even though charcoal has been found in earthworm gut material, ingestion does not necessarily indicate utilization as an energy source (Weyers and Spokas 2011). Weight loss could also be due to higher TE concentrations in the soil pore water of biochar-amended soils. Wang and Cui 2016 reported increasing stress biomarkers, including GST activity, in tissues of E. fetida exposed to arsenite, arsenate, monomethylarsonate and dimethylarsinate. Last, such weight loss could be due to higher water loss in the F-BE soil compared to the F-E soil. To our knowledge, such phenomenon was however not described in the literature.
Comparison summary of the main responses between A. longa and A. icterica exposed to the F soil, amended or not with biochar
-
Both earthworm species suffered a 4 % weight loss when exposed to the F soil.
-
This weight loss rose up to 10% (for both species) when they were exposed to the F soil amended with 5% biochar.
-
Biochar addition to the F soil did not influence TE bioavailability for A. longa.
-
Arsenic concentrations in tissues of A. icterica were lower in the biochar-amended treatment.
-
Whatever the soil exposure, Zn concentrations in A. longa differed from those of A. icterica and were four time higher.
Conclusions
The addition of poultry manure-derived biochar (2% w/w) in a contaminated technosol increased in a short-term the soil electrical conductivity as well as several trace element concentrations in the soil pore water. However, for both earthworm species, TE concentrations in earthworm bodies did not increase in the biochar-amended soil compared to the unamended one, and As concentration was even reduced in A. icterica. Out of the proxies used to determine the toxicological bioavailability, only the weight loss indicated an increased stress due to biochar amendment, while biochemical responses rather indicated no change or lower stress conditions. This study highlighted no straightforward relationship between the three TE bioavailability components. It also pinpointed the complex interactions existing between biological and physico-chemical soil components. Further investigations to characterize the soluble organic matter and its potential complexation with TE, but also the ultrastructure of such contaminated soils in presence or absence of biochar or earthworms would give relevant clues to better understand these complex interactions.
References
Adriano DC (2001) Trace elements in terrestrial environments: Biogeochemistry, bioavailability, and risks of metals, 2nd edn. Springer, Verlag, New York, NY, p 867
Ahmad M, Rajapaksha AU, Lim JE (2014) Biochar as a sorbent for contaminant management in soil and water: a review. Chemosphere 99:19–33
Arrêté du 9 août (2006) relatif aux niveaux à prendre en compte lors d’une analyse de rejets dans les eaux de surface ou de sédiments marins, estuariens ou extraits de cours d’eau ou canaux relevant respectivement des rubriques 2.2.3.0, 4.1.3.0 et 3.2.1.0 de la nomenclature annexée au décret n° 93–743 du 29mars 1993. Fr Off J 222:14082–14085
Baize D (2000) Teneurs totales en “métaux lourds” dans les sols français. Résultats généraux du programme ASPITET. Le Courrier de l’Environnement de l’INRA 39:39–54
Baize D, Deslais W, Saby N (2007) Teneurs en huit éléments traces (Cd, Cr, Cu, Hg, Ni, Pb, Se, Zn) dans les sols agricoles en France - Résultats d’une collecte de données à l'échelon national. ADEME - Gis Sol - INRA. p. 86. http://www.gissol.fr/programme/bdetm/_rapport_anademe/rapport/contents.php
Beaumelle L, Lamy I, Cheviron N, Hedde M (2014) Is there a relationship between earthworm energy reserves and metal availability after exposure to field-contaminated soils? Environ Pollut 191:182–189
Beaumelle L, Gimbert F, Hedde M, Guérin A, Lamy I (2015) Subcellular partitioning of metals in Aporrectodea caliginosa along a gradient of metal exposure in 31 field-contaminated soils. Sci Tot Environ 520:136–145
Beesley L, Dickinson NM (2011) Carbon and trace element fluxes in the pore water of an urban soil following greenwaste compost, woody and biochar amendments, inoculated with the earthworm Lumbricus terrestris. Soil Biol Biochem 43:188–196
Beesley L, Moreno-Jiménez E, Gomez-Eyles JL (2010) Effects of biochar and greenwaste compost amendments on mobility, bioavailability and toxicity of inorganic and organic contaminants in a multi-element polluted soil. Environ Pollut 158:2282–2287
Beesley L, Moreno-Jimenez E, Gomez-Eyles JL, Harris E, Robinson B, Sizmur T (2011) A review of biochars’ potential role in the remediation, revegetation and restoration of contaminated soils. Environ Pollut 159:3269–3282
Bernard L, Chapuis-Lardy L, Razafimbelo T, Razafindrakoto M, Pablo AL, Legname E, Poulain J, Bruls T, O’Donohue M, Brauman A, Chotte JL, Blanchart E (2012) Endogeic earthworms shape bacterial functional communities and affect organic matter mineralization in a tropical soil. ISME J 6:213–222
Bhadauria T, Saxena KG (2010) Role of earthworms in soil fertility maintenance through the production of biogenic structures. Appl Environ Soil Sci 2010:7 https://doi.org/10.1155/2010/816073
Blakemore RJ (2000) Ecology of earthworms under the ‘Haughley Experiment’ of organic and conventional management regimes. Biol Agric Hortic 18:141–159
Blouin M, Hodson ME, Delgado EA, Baker G, Brussar L, Butt KR, Dai J, Dendooven L, Peres G, Tondoh JE, Cluzeau D, Brun JJ (2013) A review of earthworm impact on soil function and ecosystem services. Eur J Soil Sci 64:161–182
Blouin M, Zuily-Fodil Y, Pham-Thi A-T, Laffray D, Reversat G, Pando A, Tondoh J, Lavelle P (2005) Belowground organism activities affect plant aboveground phenotype, inducing plant tolerance to parasites. Ecol Lett 8:202–208
Bot A, Benites J (2005) The importance of soil organic matter. Key to drought-resistant soil and sustained food production. FAO Soils Bulletin 80, Food and Agriculture Organization of the United Nations, Rome, p 78, http://www.fao.org/3/a-a0100e.pdf (last verified November 1, 2016)
Bouché MB (1972) Lombriciens de France: Ecologie et Systématique. Annales de Zoologie Ecologie Animale, 72-2 (HS), INRA Editions, Paris
Buss W, Mašek O, Graham M, Wüst D (2015) Inherent organic compounds in biochar--Their content, composition and potential toxic effects. J Environ Manag 156:150–157
Chan KY, Zwieten LV, Meszaros I, Downie A, Joseph S (2008) Using poultry litter biochars as soil amendments. Austral J Soil Res 46:437–444
Coq S, Barthes BG, Oliver R, Rabary B, Blanchart E (2007) Earthworm activity affects soil aggregation and organic matter dynamics according to the quality and localization of crop residues – An experimental study (Madagascar). Soil Biol Biochem 39:2119–2128
Dai J, Becquer T, Rouiller JH, Reversat G, Bernhard-Reversat F, Nahmani J, Lavelle P (2004) Heavy metal accumulation by two earthworm species and its relationship to total and DTPA-extractable metals in soils. Soil Biol Biochem 36:91–98
European Biochar http://www.european-biochar.org/en/ebc-ibi (verified on July 1, 2016)
FAO and ITPS (2015) Status of the World’s Soil Resources (SWSR) – Main Report. Food and Agriculture Organization of the United Nations and Intergovernmental Technical Panel on Soils, Rome, Italy, ISBN 978-92-5-109004-6
Fischer D, Glaser B (2012) Synergisms between Compost and Biochar for Sustainable Soil Amelioration, Management of Organic Waste, Dr. Sunil Kumar (Ed.), InTech. https://doi.org/10.5772/31200. Available from: http://www.intechopen.com/books/management-of-organic-waste/synergism-between-biochar-and-compost-for-sustainable-soil-amelioration
Folch J, Lees M, Stanley GHS (1957) A simple method for the isolation and purification of total lipids from animal tissues. J Biol Chemi 226:497–509
Fritsch C, Coeurdassier M, Faivre B, Baurand PE, Giraudoux P, van den Brink NW, Scheifler R (2012) Influence of landscape composition and diversity on contaminant flux in terrestrial food webs: a case study of trace metal transfer to European blackbirds Turdus merula. Sci. Tot. Environ 432:275–287
Gibbs HK, Salmon JM (2015) Mapping the world’s degraded lands. Appl Geogr 57:12–21
Giska I, van Gestel CA, Skip B, Laskowski R (2014) Toxicokinetics of metals in the earthworm Lumbricus rubellus exposed to natural polluted soils - relevance of laboratory tests to the field situation. Environ Pollut 190:123–132
Gomez-Eyles JL, Sizmur T, Collins CD, Hodson ME (2011) Effects of biochar and the earthworm Eisenia fetida on the bioavailability of polycyclic aromatic hydrocarbons and potentially toxic elements. Environ Pollut 159:616–622
Gregory SJ, Anderson CWN, Camps-Arbestain M, Biggs PJ, Ganley ARD, O’Sullivan JM, McManus MT (2015) Biochar in co-contaminated soil manipulates arsenic solubility and microbiological community structure, and promotes organochlorine degradation. PLoS ONE 10(4):e0125393. https://doi.org/10.1371/journal.pone.0125393
Habig WH, Pabst MJ, Jakoby WB (1974) Glutathione S-transferases the first enzymatic step in mercapturic acid formation. J Biol Chem 249:7130–7139
Hale SE, Jensen J, Jakob L, Oleszczuk P, Hartnik T, Henriksen T, Okkenhaug G, Martinsen V, Cornelissen G (2013) Short-term effect of the soil amendments activated carbon, biochar, and ferric oxyhydroxide on bacteria and invertebrates. Environ Sci Technol 47:8674–8683
Harmsen J (2007) Measuring bioavailability: from a scientific approach to standard methods. J Environ Qual 36:1420–1428
Holmstrup M, Sorensen JG, Overgaard J, Bayley M, Bindesbol AM, Slotsbo S, Fisker KV, Maraldo K, Waagner D, Labouriau R, Asmund G (2011) Body metal concentrations and glycogen reserves in earthworms (Dendrobaena octaedra) from contaminated and uncontaminated forest soil. Environ Pollut 159:190–197
Houben D, Evrard L, Sonnet P (2013) Mobility, bioavailability and pH-dependent leaching of cadmium, zinc and lead in a contaminated soil amended with biochar. Chemosphere 92:1450–1457
ISO (International Standards Organization) 11268-2 (1998) Soil quality - effects of pollutants on earthworms (Eisenia fetida) – Part 2. Determination of effects on reproduction. Geneva
ISO (International Standards Organization) 10390 (2005) Soil quality – Determination of pH. Geneva
ISO (International Standards Organization) 17402 (2008) Soil quality - Requirements and guidance for the selection and application of methods for the assessment of bioavailability of contaminants in soil and soil materials. Geneva
ISO (International Standards Organization) 17512-1 (2008) Soil quality -- Avoidance test for determining the quality of soils and effects of chemicals on behaviour -- Part 1: Test with earthworms (Eisenia fetida and Eisenia andrei). Geneva
Jégou D, Capowiez Y, Cluzeau D (2001) Interactions between earthworm species in artificial soil cores assessed through the 3D reconstruction of the burrow systems. Geoderma 102:123–137
Kidd P, Mench M, Alvarez-Lopez V, Bert V, Dimitriou I, Friesl-Hang W, Herzig R, Janssen JA, Kolbas A, Muller I, Neu S, Renella G, Ruttens A, Vangronsveld J, Puschenreiter M (2015) Agronomic practices for improving gentle remediation of trace-element contaminated soils. Int J Phytorem 17:1005–1037
Knight JA, Anderson S, Rawle JM (1972) Chemical basis of the sulfophospho-vanillin reaction for estimating total serum lipids. Clinic Chem 18:199–202
Koster M, de Groot A, Vijver M, Peijnenburg W (2006) Copper in the terrestrial environment: verification of a laboratory-derived terrestrial biotic ligand model to predict earthworm mortality with toxicity observed in field soils. Soil Biol Biochem 38:1788–1796
Kowald GR, Sturzenbaum SR, Blindauer CA (2016) Earthworm lumbricus rubellus MT-2: metal binding and protein folding of a true cadmium-MT. Int J Mol Sci 17:65. https://doi.org/10.3390/ijms17010065
Kuppusamy S, Thavamani P, Megharaj M, Venkatesvarlu K, Naidu R (2016) Agronomic and remedial benefits and risks of applying biochar to soil: current knowledge and future research directions. Environ Int 87:1–12
Lanno R, Wells J, Conder J, Bradham K, Basta N (2004) The bioavailability of chemicals in soil for earthworms. Ecotox Environ Saf 57:39–47
Lee BT, Kim KW (2008) Arsenic accumulation and toxicity in the earthworm Eisenia fetida affected by chloride and phosphate. Environ Toxicol Chemi 27:2488–2495
Lévêque T, Capowiez Y, Schreck E, Mombo S, Mazzia C, Foucault Y, Dumat (2015) Effects of historic metal(loid) pollution on earthworm communities. Sci Total Environ 511:738–746
Liebeke M, Garcia-Perez I, Anderson CJ, Lawlor AJ, Benett MH, Morris CA, Kille P, Svendsen C, Spurgeon DJ, Bundy JG (2013) Earthworms produce phytochelatins in response to arsenic. PLoS ONE 8(11):e81271. https://doi.org/10.1371/journal.pone.0081271
Liu X, Zhang A, Ji C, Joseph S, Bian R, Li L, Pan G, Paz-Ferreiro J (2013) Biochar’s effect on crop productivity and the dependence on experimental conditions-a meta-analysis of literature data. Plant Soil 373:583–594
Lu H, Zhang YY, Huang X, Wang S, Qiu R (2012) Relative distribution of Pb2+ sorption mechanisms by sludge-derived biochar. Wat Res 46:854–862
Lüscher G, Schneider MK, Lindsay A, Turnbull LA, Arndorfer M, Bailey D, Herzog F, Pointereau P, Richner N, Jeanneret P (2014) Appropriate metrics to inform farmers about species diversity.Environ Sci Pol 41:52–62
Marchand L, Nsanganwimana F, Cook BJ, Vystavna Y, Huneau F, Le Coustumer P, Lamy JB, Oustrière N, Mench M (2014) Trace element transfer from soil to leaves of macrophytes along the Jalle d’Eysines River. France and their potential use as contamination biomonitors. Ecol Ind 46:425–437
Martin NA (1986) Toxicity of pesticides to Allolobophora caliginosa (Oligochaeta, Lumbricidae). New Zeal J Agr Res 29:699–706
Menz HMM, Dixon KW, Hobbs RJ (2013) Hurdles and opportunities for landscape-scale restoration. Science 339:526–527
Morgan JE, Morgan A (1992) Heavy metal concentrations in the tissues, ingesta and faeces of ecophysiologically different earthworm species. Soil Biol Biochem 24:1691–1697
Morgan JE, Morgan A (1998) The distribution and intracellular compartmentation of metals in the endogeic earthworm Aporrectodea caliginosa sampled from an unpolluted and a metal-contaminated site. Environ Pollut 99:167–175
Morgan AJ, Stürzenbaum SR, Winters C, Grime GW, Aziz NA, Kille P (2004) Differential metallothionein expression in earthworm (Lumbricus rubellus) tissues.Ecotox Environ Saf. 57:11–19
Nahmani J, Hodson ME, Black S (2007) Effects of metals on life cycle parameters of the earthworm Eisenia fetida exposed to field contaminated, metal-polluted soils. Environ Pollut 149:44–58
Natal-da-Luz T, Ojeda G, Costa M, Pratas J, Lanno RP, Van Gestel CAM, Sousa JP (2011) Short-term changes of metal availability in soil. II: The influence of earthworm activity. Appl Soil Ecol 49:178–186
Nirola R, Megharaj M, Saint C, Aryal R, Thavamani P, Venkatesvarlu K, Naidu R, Beecham S (2016) Metal bioavailability to Eisenia fetida through copper mine dwelling animal and plant litter, a new challenge on contaminated environment remediation. Int Biodeter Biodeg 113:208–216
Oustrière N, Marchand L, Galland W, Gabbon L, Lottier N, Motelica M, Mench M (2016) Influence of biochars, compost and iron grit, alone and in combination, on copper solubility and phytotoxicity in a Cu-contaminated soil from a wood preservation site. Sci Tot Environ 566:816–825
Park JH, Choppala GK, Bolan NS, Chung JW, Chuasavathi T (2011) Biochar reduces the bioavailability and phytotoxicity of heavy metals. Plant Soil 348:439–451
Pass DA, Morgan AJ, Read DS, Field D, Weightman AJ, Kille P (2015) The effect of anthropogenic arsenic contamination on the earthworm microbiome. Environ Microbiol 17:1884–1896
Pelosi C, Lebrun M, Beaumelle L, Cheviron N, Delarue G, Nélieu S (2016) Sublethal effects of epoxiconazole on the earthworm Aporrectodea icterica. Environ Sci Pollut Res 23:3053–3061
Ponge JF (2013) Disturbances, organisms and ecosystems: a global change perspective. Ecol Evol 3:1113–1124
Pulleman MM, Six J, Uyl A, Marinissen JCY, Jongmans AG (2005) Earthworms and management affect organic matter incorporation and microaggregate formation in agricultural soils. Appl Soil Ecol 29:1–15
Qiu H, Peijnenburg WJGM, van Gestel CAM, Vijver MG (2013) Can commonly measurable traits explain differences in metal accumulation and toxicity in earthworm species? Ecotoxicology 23:21–32
R Development Core Team (2011) R: A language and environment for statistical computing. – R Foundation for Statistical Computing, Vienna, Austria, http://www.R-project.org/
Rajapaksha AU, Ahmad M, Vithanage M, Kim KR, Chang JY, Lee SS, Ok YS (2015) The role of biochar, natural iron oxides, and nanomaterials as soil amendments for immobilizing metals in shooting range soil. Environ Geochem Health 37:931–942
Rees F, Simonnot MO, Morel JL (2014) Short-term effects of biochar on soil heavy metal mobility are controlled by intra-particle diffusion and soil pH increase. Eur J Soil Sci 65:149–161
Scheifler R, Coeurdassier M, Morilhat C, Bernard N, Faivre B, Flicoteaux P, Giraudoux P, Noël M, Piotte P, Rieffel D, de Vaufleury A, Badot PM (2006) Lead concentrations in feathers and blood of common blackbirds (Turdus merula) and in earthworms inhabiting unpolluted and moderately polluted urban areas. Sci Tot Environ 371:197–205
Scheu S (2003) Effects of earthworms on plant growth: patterns and perspectives. Pedobiologia 47:846–856
Sheehan C, Kirwan L, Connolly J, Bolger T (2007) The effects of earthworm functional group diversity on earthworm community structure. Pedobiologia 50:479–487
Sims RW, Gerard BM (1999) Earthworms. FSC Publications, London
Sizmur T, Hodson ME (2009) Do earthworms impact metal mobility and availability in soil? – A review. Environ Pollut 157:1981–1989
Smith PK, Krohn RI, Hermanson GT, Mallia AK, Gartner FH, Provenzano MD, Fujimoto EK, Goeke NM, Olson BJ, Klenk DC (1985) Measurement of protein using bicinchoninic acid. Anal Biochem 150:76–85
Spurgeon D, Hopkin S (1999) Comparisons of metal accumulation and excretion kinetics in earthworms (Eisenia fetida) exposed to contaminated field and laboratory soils. Appl Soil Ecol 11:227–243
Spurgeon DJ, Svendsen C, Rimmer VR, Hopkin SP, Weeks JM (2000) Relative sensitivity of life-cycle and biomarker responses in four earthworm species exposed to zinc. Environ Toxicol Chem 19:1800–1808
Topoliantz S, Ponge JF (2005) Charcoal consumption and casting activity by Pontoscolex corethrurus (Glossoscolecidae). Appl Soil Ecol 28:217–224
Wagner A, Kopenjohann M (2014) Suitability of biochars (pyro- and hydrochars) for metal immobilization on former sewage-field soils. Eur J Soil Sci 65:139–148
Wang Z, Cui Z (2016) Accumulation, biotransformation, and multi-biomarker responses after exposure to arsenic species in the earthworm Eisenia fetida. Toxicol Res 5:500–510
Weyers SL, Spokas KA (2011) Impact of biochar on earthworm populations: a review. Appl Environ Soil Sci 2011:12Article ID 541592, https://doi.org/10.1155/2011/541592
Yasmin S, D’Souza D (2010) Effects of pesticides on the growth and reproduction of earthworm: a review. Appl Environ Soil Sci 2010:9. Article ID 678360, https://doi.org/10.1155/2010/678360
Zhang Z, Solaiman Z, Meney K, Murphy D, Rengel Z (2013a) Biochars immobilize soil cadmium, but do not improve growth of emergent wetland species Juncus subsecundus in cadmium-contaminated soil. J Soils Sedim 13:140–151
Zhang X, Wang H, He L, Lu K, Sarmah A, Li J, Bolan NS, Pei J, Huang H (2013b) Using biochar for remediation of soils contaminated with heavy metals and organic pollutants. Environ Sci Pollut Res 20:8472–8483
Acknowledgements
Authors are members of INRA’s ecotoxicologist network (http://www6.inra.fr/ecotox). Authors thank the Biochem-Env platform (UMR 1402 ECOSYS) for measuring soil enzymatic activities and energy resources. Biochem-Env is a service of the “Investment for the Future” infrastructure AnaEE-France, overseen by the French National Research Agency (ANR) (ANR-11-INBS-0001). Authors are grateful to A. Trouvé, S. Breuil, E. Ollivier, N. Oustrière and W. Galland for their technical help. We also thank Dr. V. Bert (INERIS) and les Voies Navigables de France for providing the Phytosed-technosol, and C. Grosbellet from Florentaise (La Grande Gâcherie, 44850 Saint-Mars du Désert, France) for the biochar. Last but not least, authors warmly thank Dr. S. Weyers for her valuable comments on the manuscript. Dr M Mench acknowledged the support of the ERA-Net FACCE-SURPLUS (project INTENSE).
Author information
Authors and Affiliations
Corresponding author
Ethics declarations
Conflict of interest
The authors declare that they have no competing interests.
Informed consent
Informed consent was obtained from all individual participants included in the study.
Electronic supplementary material
Rights and permissions
About this article
Cite this article
Marchand, L., Brunel-Muguet, S., Lamy, I. et al. Modulation of trace element bioavailability for two earthworm species after biochar amendment into a contaminated technosol. Ecotoxicology 26, 1378–1391 (2017). https://doi.org/10.1007/s10646-017-1862-8
Accepted:
Published:
Issue Date:
DOI: https://doi.org/10.1007/s10646-017-1862-8