Abstract
Exotic earthworm impacts on temperate forest soils are influenced by earthworm community composition and are likely constrained by the degree of organic matter redistribution following earthworm introductions across different soil types; however, the relative importance of these factors remains unknown. We examined how exotic earthworm communities affected leaf litter carbon (C) and nitrogen (N) mineralization and transport in two Spodosols with contrasting textures and organic matter contents. In reconstructed soil mesocosms, we measured organic C pools, quantified 13C and 15N transport from isotopically labeled red maple (Acer rubrum) leaf litter, and linked leaf litter redistribution to sub-surface burrow system structures following 150-day incubations. Transport of C and N from surface litter into soil was greatest with multi-species earthworm communities, and A-horizon and burrow pools functioned as dominant sinks for this material. Litter-derived C:N recovery ratios of soil pools revealed higher retention of litter-derived N over litter-derived C; recovery of litter N (mg 15N m−2) transported from surface litter was greater in the sandy loam (98.2 ± 2.73 %) than in the sandy soil (66.2 ± 4.92 %) following earthworm community additions. Earthworm biomass was as a minor sink for litter C (mg 13C m−2) and N transported from surface litter (0.56 ± 0.13 and 2.26 ± 0.31 %, respectively). Recovery of litter-derived C and N in earthworm biomass increased with the degree of direct leaf litter consumption (A. trapezoides < E. fetida < L. terrestris). Surface-dwelling epigeic and mineral-soil dwelling endogeic species produced burrow systems with the highest volume, surface connectivity, and density in the A-horizon; these properties were associated with greater CO2 losses and with greater litter C and N transport into A-horizons and burrows. Burrow systems with high continuity and large burrows produced by vertical-burrowing anecic species were associated with greater litter C and N transport into B-horizons, and greater dissolved organic C leaching losses. This study shows that the degree of organic matter redistribution in temperate forest soils following earthworm introductions is directly related to earthworm community composition, while the preferential retention of N over C and the potential stabilization of this material is determined by soil type.
Similar content being viewed by others
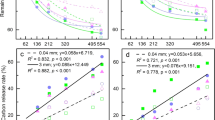
Explore related subjects
Discover the latest articles, news and stories from top researchers in related subjects.Avoid common mistakes on your manuscript.
Introduction
Soil C dynamics in temperate forests are of particular interest because soil organic matter (SOM) in these ecosystems functions as a long-term C sink for atmospheric CO2 (Gaudinski et al. 2000, Gough et al. 2008). However, the stability and size of this C sink is vulnerable to disturbances that can destabilize and alter SOM distributions within soil profiles. For example, large changes in soil C stocks and composition have been linked to exotic earthworm species introductions into previously earthworm-free temperate forests in North America (Bohlen et al. 2004a). These shifts include reductions in surface soil C stocks (Alban and Berry 1994; Burtelow et al. 1998; Bohlen et al. 2004b; Wironen and Moore 2006; Fahey et al. 2012), related shifts in soil C depth distribution (Burtelow et al. 1998; Bohlen et al. 2004b; Straube et al. 2009; Fahey et al. 2013), greater CO2 efflux (McLean and Parkinson 1997; Li et al. 2002; Fisk et al. 2004; Eisenhauer et al. 2007), and increased dissolved organic C (DOC) losses (Scheu and Parkinson 1994; McInerney and Bolger 2000; Bohlen et al. 2004b). The magnitude of these shifts varies widely across studies, and this variable response has been attributed to differences in soil edaphic properties, land use history, tree species composition, and earthworm community composition. Further, the long-term effects of belowground disturbances on forest soil C dynamics are largely unknown, in part because few controlled experiments have considered both soil C budgets and the nature of the soil C remaining after earthworm introductions, and few studies have considered multiple edaphic factors (e.g., Crumsey et al. 2013a; Zhang et al. 2013).
Earthworm impacts on SOM dynamics may be constrained by the pool size of potentially mineralizable C in earthworm-invaded soils (Zhang et al. 2013). Within temperate forest soils, the pool size of mineralizable C has been in part related to leaf litter incorporation into soils and redistribution of decomposition products within soil profiles (Fahey et al. 2013). The pool size of mineralizable C in forest soils is also determined by stabilization mechanisms that vary in strength with soil physical and chemical properties (e.g., mineralogy, redox, pH, texture) (Sollins et al. 1996; Torn et al. 1997; Swanston et al. 2005; Suárez et al. 2006b). Further, earthworm distributions vary spatially (within soil depth profiles and across ecosystems) as a function of soil properties that include C concentration, and as a function of varying dispersal abilities of species belonging to different ecological groups (Hughes et al. 1996; Hale et al. 2005a; Holdsworth et al. 2007; Crumsey et al. 2013b). Consequently, the impact of earthworms on soil C dynamics needs to be considered alongside the effects of soil texture, particularly those with differing physical and chemical properties and that co-occur in earthworm-invaded forest ecosystems.
Finally, the biogeochemical cycling of C in temperate forest soils is inextricably linked to that of N through multiple processes, such that aboveground net primary productivity and belowground soil C storage are generally constrained by soil N availability (Zak et al. 1989; Nadelhoffer et al. 1999a; Waldrop et al. 2004; Nave et al. 2011). Exotic earthworm activity in temperate forests has been shown to alter the distribution and turnover of N, albeit to a lesser extent than that of C in some cases. Shifts include increased bulk N pool sizes, net N mineralization and nitrification rates, and total organic N leaching losses (Alban and Berry 1994; Burtelow et al. 1998; Bohlen et al. 2004b; Costello and Lamberti 2009; Fahey et al. 2013). Therefore, it is necessary to assess the influences of earthworm activity on soil C budgets by determining how both litter C and N inputs are distributed within forest soils following exotic earthworm introductions.
In this study, we used 13C- and 15N-labeled leaf litter additions to mesocosms to examine how mono-specific and multi-species earthworm communities control leaf litter C and N incorporation into forest soils of two different textures and organic matter contents. In community treatments, we placed emphasis on interspecific interactions involving an endogeic species that strongly influences soil macroporosity and organic matter stabilization through subsurface cast and horizontal burrow production (Jégou et al. 1997, 2000; Marhan and Scheu 2006; Crumsey et al. 2013a), and which has been shown to interact with epigeic and anecic species with differential effects on soil nutrient cycling along earthworm invasion chronosequences (e.g., Hale et al. 2005a; Resner et al. 2014). We addressed three hypotheses:
-
(1)
The total amount of litter C and N transported from surface litter into soils would increase with earthworm additions to soils, and the magnitude of this increase would be greatest with direct leaf litter consumption by anecic species and sub-surface burrow production.
-
(2)
The amount of litter-derived C and N recovered in soil would be texture-dependent with increasing clay content showing higher levels of litter-derived C and N retention due to inherent soil differences in C stabilization capacity.
-
(3)
Patterns of litter-derived C and N redistribution and loss would be directly related to the volume and continuity of sub-surface burrow systems produced through earthworm activity due to organic matter accumulation and enhanced gaseous and dissolved C and N transport.
Results are presented from a 150-day mesocosm experiment in which earthworm species of three functional groups were combined in a partial factorial design, and earthworm community treatments were replicated in reconstructed sandy and sandy loam Spodosols.
Methods
Study area
This study was conducted at the University of Michigan Biological Station in northern Michigan, US (45°35.5′N, 84°43′W), where secondary successional forests are dominated by bigtooth aspen (Populus grandidentata), with northern red oak (Quercus rubra), red maple (Acer rubrum), paper birch (Betula papyrifera), American beech (Fagus grandifolia), and eastern white pine (Pinus strobus) as co–dominants (Curtis et al. 2005). Earthworm communities are dominated by five species representing different ecological groups: Dendrobaena octaedra (Savigny), Aporrectodea caliginosa (Savigny), Aporrectodea trapezoides (Dugès), Lumbricus rubellus (Hoffmeister), and Lumbricus terrestris (Linnaeus). Earthworm community biomass ranges from 2 ± 0 to 47 ± 15 g m2 (fresh weight), and abundance ranges from 7 ± 1 to 92 ± 56 individuals m2 across upland soils (Crumsey et al. 2013b). Forests lie on well-drained Spodosols classified as mixed, frigid Entic Haplorthods of the Rubicon series (92.9 % sand, 6.5 % silt, 0.6 % clay), and mixed Alfic Haplorthod of the Alcona series (69.2 % sand, 14.2 % silt, 16.6 % clay) (National Resources Conservation Service 1991).
Experimental design
We conducted a mesocosm experiment from May to October 2011 in a belowground laboratory (Lussenhop et al. 1991) at the University of Michigan Biological Station (UMBS; Appendix A, supplementary material), using earthworm community treatments and no-earthworm controls in uniform, reconstructed soil profiles. We used two dominant forest soil types: (1) the coarse-textured sandy Entic Haplorthod and (2) the finer-textured sandy loam Alfic Haplorthod. Mesocosms were contained in 20-L plastic buckets, and were 28 cm in diameter and 30 cm in depth. Soil profiles were constructed by adding 25 kg (fresh weight) of sieved (2 mm) and homogenized B-horizon soil packed to a bulk density of 1.5 g cm−3, 25 cm in depth, plus 5 kg (fresh weight) of sieved and homogenized A-horizon soil packed to a bulk density of 1.3 g cm−3, 5 cm in depth. Soil moisture was maintained at field capacity (~20 % v/v) with 500 mL de-ionized water additions as required. Zero-tension lysimeters installed below each mesocosm allowed for drainage.
Earthworm species of different ecological groups included: Lumbricus terrestris [anecic = leaf litter feeding, vertical burrowing], Eisenia fetida [epigeic = leaf litter feeding, surface–dwelling], and Aporrectodea trapezoides [endogeic = mineral soil feeding and dwelling]. E. fetida, a peregrine species that inhabits organic-rich soils (Tiunov et al. 2006), was used in place of its ecological equivalent D. octaedra due to the low biomass of D. octaedra available in local earthworm communities (Crumsey et al. 2013b). To evaluate intraspecific interactions, we implemented a partial factorial experimental design where treatments (hereafter capitalized) included (a) monocultures: Epigeic and Anecic; and (b) mixed treatments: Epigeic × Endogeic, Anecic × Endogeic, and All Species. To evaluate the interactions of soil texture with earthworm community impacts, the All Species treatment was replicated in the finer-textured sandy loam. Total earthworm biomass was 20 ± 0.5 g (fresh weight) per mesocosm. Thus, earthworm biomass added was 10 ± 0.5 g of each species in two-species treatments and 6.5 ± 0.5 g for each species in the All Species treatments.
Acer rubrum (isotopic tracer) 13C and 15N enrichment and mixed leaf litter additions
In our previous study with the sandy Spodosol used here, A. rubrum litter was consumed at higher rates than leaf litter from other tree species dominating this forest site (Crumsey et al. 2013a). Therefore, we used 13C- and 15N-enriched A. rubrum litter as an isotopic tracer to quantify C and N transport from the leaf litter layer into mineral soil horizons. A. rubrum seedlings were grown in a temperature-controlled chamber at Queens College, City University of New York, in which seedlings were labeled weekly with 13C-enriched CO2 for 18 weeks, and with15N-enriched NH4Cl and KNO3 fertilizer (liquid) weekly for 21 weeks (described in Bird and Torn 2006).
Senesced leaves were dried at 60 °C and included in mixed leaf litter additions scaled in composition and mass (128 g C m−2, 2 g N m−2) from area-normalized leaf litter data of the UMBS AmeriFlux site in 2008 (C. S. Vogel, unpublished data). In total, 16.8 g mixed leaf litter from dominant canopy tree species were added to each mesocosm at the start of incubation as follows: 27 % dual-labeled A. rubrum, 40 % Populus grandidentata, 21 % Quercus rubra, 7 % Betula papyrifera, 4 % Fagus grandifolia, and 1 % Pinus strobus. The C and N chemistry of dual-labeled A. rubrum litter was as follows: 41.9 % C, 1.06 % N, 4.48 atom % 13C, and 5.86 atom % 15N, such that the initial isotopic mass added to each mesocosm was 1382 mg 13C m−2 and 45.8 mg 15N m−2.
Mesocosm sampling, C and N content, isotopic analysis
Mesocosms were destructively harvested by first collecting intact leaf litter remaining on the soil surface. Soils were then excavated by removing the A-horizon (5 cm depth), followed by removal of B-horizon in 5 cm to 10 cm depth increments separated into burrow and bulk soil (i.e., soil not visibly altered by earthworm burrowing activity or ingestion). Burrow soils were the sum of vertical burrows created by anecic species, galleries created by endogeic species, and casts produced within these structures. Organic matter accumulation resulted in burrow soils that were darker in color and that showed greater aggregation than unaltered bulk B-horizon soils (Brown et al. 2000); this allowed for the physical separation of burrow and bulk soil mass in the 5–25 cm layer of each mesocosm. Separation of burrow and bulk soil in the A-horizon was not feasible due to high-density burrow networks created across all earthworm treatments (Fig. 1).
Three-dimensional reconstructions of earthworm community burrow systems; imaged by X-ray CT. Color gradations used for 3-D rendering, yellow for the foreground to blue for the background, represent distance of burrows from the viewer’s perspective. Earthworm species of different functional groups included: L. terrestris [Anecic = litter feeding, vertical burrowing], A. trapezoides [Endogeic = mineral soil feeding and dwelling], and E. fetida [Epigeic = litter feeding, surface–dwelling]. Soil depth (cm) and bulk density (BD) of the A-horizon (AH) and B-horizon (BH) were uniform across treatments. Mean values (n = 6) of burrow system continuity, volume, and size distribution quantified after reconstructions are shown in Table B1, supplementary material. (Color figure online)
Recovered earthworms were weighed fresh, frozen at −80 °C, freeze-dried, and weighed again to obtain dry weight correction factors. Litter and soil subsamples were weighed fresh, dried at 60 °C, and weighed again to obtain dry weight correction factors. Percent C, percent N, and stable isotope ratios (expressed as δ13C and δ15N) were measured on pulverized samples by continuous flow isotope ratio mass spectrometry (Thermo Finnigan Delta Plus XL) after sample combustion to CO2 and N2 at 1000 °C by an on-line elemental analyzer (Costech Elemental Analyzer 4010). Instrument precision was verified by repeated analysis of internal laboratory standards including acetanilide, caffeine, and albumin. Instrument error was ± 0.16 ‰ for δ15N, and ± 0.19 ‰ for δ13C. Initial C and N properties of litter, soil, and earthworm pools are shown in Table A1, supplementary material.
Acer rubrum 13C and 15N recoveries in soils and earthworm biomass
We calculated A. rubrum litter 13C and 15N transport into soil and earthworm biomass pools using elemental pool sizes, and increases in 13C and 15N content of pools following leaf litter additions (i.e., 13C and 15N excess); this approach assumes no changes over time in 13C and 15N natural abundance in the pools considered (Nadelhoffer and Fry 1994; Fahey et al. 2011). A. rubrum 13C and 15N pools were calculated at time zero and at the end of incubations as the product of dry weight, C and N content, and isotopic atom % enrichments of 13C and 15N. Large differences in the δ13C and δ15N values between non-labeled pools and dual-labeled A. rubrum litter minimized the effects of isotopic fractionation on our estimates of A. rubrum 13C and 15N recoveries. In turn, narrow ranges of δ13C and δ15N values in non-labeled soil and earthworm biomass pools facilitated detection of excess 13C and 15N incorporated into these pools via dual-labeled A. rubrum litter decomposition and redistribution (Table A1, supplementary material).
We calculated percent recoveries (Eq. 1) of A. rubrum litter C and N mass (m Ar ; mg m−2) transported from the litter pool during the 150-day incubation (initial mass to final mass; i to f) into soil and earthworm biomass pools (m pool ; mg m−2) (modified from Hayes 2004); this approach accounts for variation in A. rubrum decomposition among individual mesocosms by using differences in tracer masses added to mesocosms vs. tracer masses remaining in litter (the denominator in Eq. 1) to estimate redistributed tracer masses. The percent recovery was calculated as the quotient of excess heavy isotope (H = 13C or 15N) recovered in each pool and the amount of A. rubrum tracer mass transported from the litter pool using the following equation:
where percent recovery (subscript Ar.rec) is calculated for each element (X = C or N), H F pool = fractional abundance of heavy isotope calculated as 13C/(12C + 13C) or 15N/(14N + 15N) that is equivalent to atom % (HX/100) in a pool at the end of incubations, H F ref = fractional abundance of heavy isotope in a soil or earthworm biomass pool at the start of incubations, and H F Ar = fractional abundance of heavy isotope in A. rubrum litter. Though cumulative CO2 and DOC losses were measured (see Appendix B, supplementary material for details), the isotopic composition of C and N loss pools were not measured in this study. Thus, A. rubrum 13C and 15N not recovered in leaf litter, soil, or earthworm biomass was attributed to litter-derived gaseous (CO2 and NOx) exports and leachate (dissolved C and N) losses.
Finally, we calculated the amounts of total A. rubrum C and N transported into soil pools and earthworm biomass, and the amounts attributed to gaseous and leachate losses, using the 13C and 15N excess recovered in each pool and isotopic mass balance. A litter-derived C:N recovery ratio was then calculated as the quotient of A. rubrum-derived C to A. rubrum-derived N for soil pools and recovered earthworm biomass. This ratio uses the isotope tracers to describe the net transport efficiency of litter-derived C to litter-derived N within our mesocosms (Bird et al. 2008).
3D reconstruction and quantification of burrow systems
Prior to mesocosm harvesting, soils containing earthworm treatments were imaged using X-ray computed tomography (Discovery CT 750 HD scanner, 120 kV, 110 mA, 9.04 s, 0.969:1 pitch, 1.25 mm slice interval, 1.25 mm slice thickness, 40 cm field of view, bone reconstruction filter) at the University of Michigan School of Radiology. Two-dimensional images (horizontal sections of soil every 1.25 mm, 0.78 mm X and Y resolution) were produced in a 16-bit DICOM format with grey-levels expressed in Hounsfield values that relate to the attenuation of X-ray beams passed through soils. Using ImageJ (available online Footnote 1), the depth of each image was reduced to an 8-bit format. Grey-level histograms of 8-bit images were bimodal, with a large peak (grey values) corresponding to the soil matrix and a narrow peak (black values) corresponding to voids and thus to macropores produced by earthworm burrowing activity (Capowiez et al. 1998).
Volumetric information on macropores in 2-D images was translated into 3-D skeletons by identifying macropore centroids, and linking those that overlap between successive images (Pierret et al. 2002, Bastardie et al. 2005). A burrow was defined as a set of connected segments (joined centroids of two overlapping macropores) and the burrow system as the set of burrows in a mesocosm. From this information, we calculated total macroporosity (i.e., volume, cm3), burrow volume in the A-horizon, surface connectivity (burrow volume directly connected to the soil surface), and burrow size distribution (0.1–0.17, 0.17–0.34, and >0.34 cm2). To characterize burrow system continuity, horizontal planes were defined at four depths (0–15 %, 15–25 %, 25–50 %, and >50 % of core length); the burrow number connecting each successive plane was summed for each mesocosm (Capowiez et al. 2011; Crumsey et al. 2013a). Burrow system characterization was conducted at the French National Institute for Agricultural Research.
Statistical analyses
The effects of earthworm community composition and soil texture on litter-derived C and N redistribution were determined by linear mixed-effects models, using treatments, pools (soil horizons: A-horizon, B-horizon, burrow soil; earthworm biomass: L. terrestris, E. fetida, A. trapezoides), and elements (C and N) as fixed effects and treatment replicates (i.e., individual mesocosms) as random effects. Least square means were used to compare litter-derived C and N redistribution within pools across treatments. To characterize relationships between soil chemistry and burrow system data, we first evaluated correlations among burrow systems and soil chemistry variables using Spearman rank correlation coefficients (ρ). Associations between soil and burrow system variables were then characterized using co-inertia analysis (CoIA), which identifies relationships between two data matrices first transformed by principal component analysis (Dray et al. 2003). Significance of the CoIA was assessed by Monte Carlo permutation tests (999 permutations; α = 0.05). Statistics were done in R v3.1.20 (R Development Core Team 2013) on RStudio v 0.98.1060, using the packages nlme (Pinheiro et al. 2015), lsmeans (Lenth and Hervà 2015), Hmisc (Harrell 2012), and ade4 (Dray and Dufour 2007).
Results
Losses of A. rubrum 13C and 15N from the surface litter
Leaf litter consumption by earthworm communities accelerated the loss of A. rubrum 13C and 15N loss from the litter layer compared to soils without earthworm additions over the 150-day incubations (Table 1). Without earthworms, the loss of A. rubrum 13C from the litter layer in the sandy soil was 54 % of the initial 13C pool, and was 74 % in the finer-textured sandy loam (Table 1). Additions of epigeic and endogeic species increased mean loss of A. rubrum 13C from the litter layer from 54 to 62 %. However, the addition of anecic species (across Anecic, Anecic × Endogeic, and All Species treatments) increased the loss of A. rubrum 13C to 77–95 % of that applied (Table 1). Without earthworms, A. rubrum 15N loss in the sandy soil was 43 % of the initial 15N pool, and was 77 % in the finer-textured sandy loam (Table 1). In contrast to the loss of A. rubrum 13C, additions of epigeic and endogeic earthworms had no effect on the loss of A. rubrum 15N from the litter pool; earthworm community additions that included anecic species increased A. rubrum 15N losses to 75–95 % of the initial 15N pool. A. rubrum litter redistribution, the decomposition of this material, and subsequent assimilation into SOM and earthworm biomass resulted in the isotopic enrichments of soil and earthworm biomass pools (Tables C1 and C2, supplementary material).
Earthworm biomass remaining after the 150-day incubations was a small sink for A. rubrum 13C and 15N; this was likely due to low assimilation rates in living biomass and biomass loss over 150-day incubations. Biomass loss across treatments was 44 ± 2 % for A. trapezoides, 78 ± 3 % for E. fetida, and 42 ± 10 % for L. terrestris (mean ± SE, n = 4). Litter-derived 13C and 15N recovery in earthworm biomass ranged from 0.15 – 0.84 % of applied 13C and 0.98–2.9 % of applied 15N (Table 1). Overall, A. rubrum 13C and 15N recovery in earthworm biomass increased with the degree of direct leaf litter consumption in the following order: A. trapezoides < E. fetida < L. terrestris (Table C3, supplementary material).
Soil recovery of A. rubrum-derived C and N
We used isotopic mass balance and the 13C and 15N excess recovered in each pool to calculate (1) the amounts of total A. rubrum C and N transported from the litter pool into soil and earthworm biomass (Table C3, supplementary material), and (2) the influence of treatment on the relative recoveries and losses of A. rubrum-derived C and N across soil and earthworm biomass pools (Table 2). A. rubrum 13C and 15N recoveries were used to determine A. rubrum-derived C and N redistributed throughout soil profiles, assimilated into earthworm biomass, and lost from the system.
Total recoveries of A. rubrum C and N transported from surface litter into soil pools were significantly higher in the finer-textured sandy loam than in the sandy soil, relatively higher for litter-derived N than for C, and increased with earthworm community additions in both soil textures (Fig. 2; Table C4, supplementary material). Of the initial A. rubrum-derived C applied to soil surfaces (30.8 g C m−2), 3 % was recovered in the sandy soil and 53 % was recovered in the sandy loam soil (Table C4, supplementary material). In contrast, additions of earthworms to sandy soils increased the recovery of A. rubrum C by 4–35 % of that applied to the litter layer and increased A. rubrum C recovery by 33 % in the finer-textured sandy loam (Table C4, supplementary material). Of the initial A. rubrum-derived N applied (0.78 g N m−2), we recovered 8 % in the sandy soil and 38 % in the sandy loam soil. Earthworm additions to sandy soils increased total A. rubrum N recovery in soil by 13–73 % and increased total A. rubrum N recovery by 58 % in the sandy loam.
a Litter-derived C (g C m−2) and b litter-derived N (mg N m−2) retention in the leaf litter (white bars), recovery in soil and earthworm biomass pools (calculated from 13C and 15N excess; grey bars), and gaseous/leachate losses (black bars) after 150-day incubations. Uppercase letters represent significant differences in total A. rubrum C and N recovery (summed across soil and earthworm biomass pools) across treatments (ANOVA, Tukey HSD post hoc test; P < 0.05). Lowercase letters represent significant differences in A. rubrum C and N redistribution in pools across treatments (asterisks represent significantly higher isotopic recovery in B-horizon pools; ANOVA, Tukey HSD post hoc test; P < 0.05). Means (±1 S.E.) are shown in Table C4, supplementary material
A. rubrum C and N redistribution from the surface litter to the soil also varied with earthworm community composition in the sandy soil and across soil types (Fig. 2; Table C4, supplementary material). Epigeic species additions resulted in statistically similar levels of A. rubrum-derived C and N recovery to those observed in no-earthworm controls. However, the addition of both endogeic and epigeic species to the sandy soil increased total A. rubrum C recovery in soil by 16 % and increased total A. rubrum N recovery by 28 %. The inclusion of anecic species in earthworm community additions to the sandy soil increased total A. rubrum C recovery in soil by 21–35 % and increased total A. rubrum N recovery by 44–73 %, with the highest recoveries observed with the added inclusion of endogeic species. B-horizons of the sandy loam showed significantly higher recovery of A. rubrum C (39 %) than B-horizons of the sandy soil (1 %) irrespective of earthworm community additions (Fig. 2); likely indicating sub-soil retention of dissolved A. rubrum-derived C moving through sandy loam profiles.
A. rubrum C and N gaseous and leachate losses
A. rubrum-derived C and N not recovered in litter, soil, or earthworm biomass was attributed to gaseous (CO2 and NOx) and leachate (dissolved C and N) losses (Fig. 2; Table C4, supplementary material). Total CO2 losses from the system were orders of magnitude higher than losses of DOC in both sandy and sandy loam soils (Fig. B1, supplementary material), such that gaseous losses via CO2 were the dominant export pathway of A. rubrum-derived C loss from our mesocosms. Without earthworms, A. rubrum C loss from the litter–soil system was 42 % of that applied in the sandy soil, and 17 % of that applied in the sandy loam soil. Litter losses in sandy soil increased by 13–21 % of the initial C pool with earthworm community additions that included anecic species, but were similar between no-earthworm controls and earthworm community additions excluding anecic species (Epigeic and Epigeic × Endogeic treatments). In contrast to the sandy soil, earthworm community additions in the sandy loam reduced A. rubrum C loss from the litter–soil system to 10 % of that applied compared with the no-earthworm control.
Without earthworms, total A. rubrum N losses from the litter–soil system were similar in magnitude (% of initial pool) to those of A. rubrum C loss; A. rubrum N loss was 24 % of that applied in the sandy soil, and was 36 % of that applied in the sandy loam (Table C4, supplementary material). A. rubrum N losses from the litter–soil system declined across all earthworm community treatments, with the sharpest declines observed with the inclusion of endogeic species in earthworm community additions (−15 to +9 % of the initial N pool). Possible differences in gaseous vs. leachate losses of N could not be inferred, as N losses were not directly measured in this study.
A. rubrum C:N recovery ratios in soil and earthworm biomass
We measured the ratio of A. rubrum C and N across pools to determine relative differences in the redistribution and retention of litter-derived C and N among treatments (Fig. 3a; Table C5, supplementary material). Litter-derived C:N ratios in sandy A-horizon (9–15), B-horizon (1–4), and burrow soils (10–15) were significantly lower than corresponding bulk soil C:N ratios in all except the Epigeic × Endogeic treatment. B-horizons of the sandy soil showed lower litter-derived C:N recovery ratios than A-horizon and burrow soils (Table C5, supplementary material). In contrast, sandy loam B-horizons showed higher bulk soil C:N and litter-derived C:N ratios (46–49 and 30–42, respectively) than A-horizon and burrow soils. Litter-derived C:N ratios that were lower than the initial A. rubrum C:N ratio of 39.5 and bulk soil C:N ratios were consistent across pools in both soil types.
Comparisons between bulk C:N ratios (black symbols) and litter-derived C:N recovery ratios (grey symbols) across a soil pools: A-horizon, B-horizon, and Burrow soil, and b earthworm species biomass: A. trapezoides (Endogeic = mineral soil feeding and dwelling), E. fetida (Epigeic = litter feeding, surface–dwelling), and L. terrestris (Anecic = litter feeding, vertical burrowing). Points represent means and error bars show 95 % confidence intervals. Significant differences in bulk soil C:N ratios and litter-derived C:N ratios within treatments are shown in Table C5, supplementary material. The initial litter C:N of A. rubrum litter applied was 39.5
Litter-derived C:N ratios (10–19) within earthworm biomass were higher than those observed for bulk earthworm biomass (Fig. 3b, Table C5, supplementary material), and decreased with the degree of surface leaf litter feeding (A. trapezoides [endogeic] > E. fetida [epigeic] > L. terrestris [anecic]; Fig. 3b). Litter-derived C:N ratios in mixed species treatments were significantly lower than those observed in species monocultures for L. terrestris biomass; litter-derived C:N ratios for E. fetida and A. trapezoides biomass were similar across all treatments (Table C5, supplementary material). Overall C:N ratios of earthworm biomass were consistent across species and treatments (4 ± 0.06; Table C5, supplementary material).
Earthworm burrow system structure and litter redistribution
The magnitude of litter-derived C and N redistribution was directly linked to the volume and continuity of sub-surface burrow systems, which differed in relation to earthworm community composition (Fig. 4; Table B1, supplementary material). For the co-inertia analysis, we retained variables with Spearman rank correlation coefficients (ρ) less than 0.80 (Tables D1 and D2, supplementary material). We then evaluated variable associations along the first two co-inertia axes (eigenvalues: F1 = 4.22; F2 = 1.76), which together explained 90.5 % of the total variability in the soil and burrow system data co-structure (Monte Carlo permutation tests, P = 0.002; Fig. 5). Burrow systems with the greatest volume, surface connectivity, and densest networks of small burrows (<0.34 cm2) in the A-horizon were most associated with endogeic and epigeic species, and strongly related to A. rubrum 13C loss as CO2 from the litter–soil system, A. rubrum 13C recovery in the A-horizon, and high A-horizon bulk C mass. Burrow systems of low to intermediate continuity (burrows extending up to 7.5 cm below soil surface, 0–25 % of mesocosm length) were most associated with anecic species, and strongly related to the magnitude of A. rubrum 13C and 15N loss from the litter pool, and to DOC losses over the 150-day incubations.
Relationship between burrow system volume and burrow surface connectivity (cm3) across earthworm community treatments (ρ = 0.90, P < 0.001). Mean values (±1 S.E.) of burrow system continuity, volume, and size distribution and significant differences in burrow system properties across treatments are shown in Table B1, supplementary material
Relationships between burrow system properties (dashed arrows) and A. rubrum tracer redistribution (solid arrows) according to relative positions on the Fl × F2 co-inertia plane. Burrow (BR) system structure measures: macroporosity (total volume), size distribution (0.17 to <0.34 cm2 and >0.34 cm2), and continuity (burrows continuous through 0–15, 15–25, 25–50, and >50 % of core length). Tracer redistribution measures: A. rubrum (Maple) 13C transport from the soil surface and 13C gains in soil pools (A-horizon, AH; B-horizon, BH; Burrow, BR). Bulk C distribution measures: CO2 loss, DOC loss, and C mass across soil pools. Co-inertia axis eigenvalues: F1 = 4.22; F2 = 0.76 (Monte Carlo Tests, P = 0.002). Variables not shown were highly correlated to those included in the co-inertia analysis (ρ > 0.80; Tables D1 and D2, supplementary material)
Discussion
Acer rubrum C and N recoveries in soil pools
Our results showed large differences in the effects of soil texture and earthworm species in controlling degradation, transport, and redistribution of litter-derived C and N. Recoveries of A. rubrum C and N were greatest in A-horizon and burrow pools and were higher within the sandy loam soil with additions of all three earthworm species, and within the sandy soil containing both endogeic and anecic earthworm species. There are two likely mechanisms for variations in rates of leaf litter transport into soils among earthworm community treatments. These mechanisms include: (1) fundamental differences in the physical redistribution of surface litter by earthworm feeding and burrowing behavior (Jégou et al. 2000; Capowiez et al. 2011), and (2) eluviation of isotopically enriched dissolved organic matter into deeper soil layers (Nadelhoffer and Fry 1988; Garten et al. 2008). Accelerated leaf litter mass transport from the soil surface observed in this study is likely driven by direct litter consumption by anecic species, while increased transport to A-horizon and burrow soils is enhanced through the bioturbation of mineral soils by endogeic species (Hendriksen 1990; Suárez et al. 2006a; Holdsworth et al. 2008; Fahey et al. 2011, 2013).
Patterns of total litter C and N isotope recoveries were comparable to those previously reported for transfers of C and N from leaf litter into soil pools of northern temperate forests (Nadelhoffer et al. 1999b; Zeller et al. 2001; Fahey et al. 2011). For example, using sugar maple litter double-labeled with 13C and 15N, Fahey et al. (2011) quantified fluxes of C and N between litter and northern hardwood forest soil, and observed higher recovery of litter 15N in soil than litter 13C (over 90 % higher for N than C). Previous work also suggests C and N dynamics in earthworm-invaded soils shift over time. Two years following the application of 13C- and 15N-labeled leaf litter to field plots in a maple-dominated temperate forest, earthworm-invaded soils showed lower total 13C and 15N recovery than earthworm-free soils, but a greater concentration of litter incorporated into macro- and micro-aggregates (Fahey et al. 2013); these trends were attributed to earthworm over-wintering activity, subsequent losses in forest floor mass, and accelerated leaf litter processing in earthworm-invaded soils. Together, our results and those of previous studies (e.g., Zhang et al. 2013) suggest short-term increases in leaf litter transport into soil pools and possible protection of organic matter in earthworm burrows and surface soils.
Notably, recoveries of A. rubrum-C in sandy loam B-horizons were comparable to A. rubrum C recoveries summed across A-horizon and burrow soils of both soil types. High A. rubrum C recovery in sandy loam B-horizons, and negligible recovery in B-horizons of sandy soils, was independent of earthworm community additions. These results indicate eluviation of isotopically enriched dissolved organic matter into deeper soil layers, and subsequent retention of A. rubrum C in clay-rich subsoils. Together, higher gaseous and leachate C losses (Appendix A, supplementary material) and greater litter-derived C retention in sandy loam soils than in sandy soils are consistent with a larger labile C pool (i.e., humified, low density organic material) subject to increased rates of microbial degradation (e.g., Gaudinski et al. 2000; Bird et al. 2008).
Acer rubrum C and N recoveries in earthworm biomass
Earthworm biomass was a minor sink for A. rubrum litter, which may be due to low assimilation efficiencies by the earthworms (Curry and Schmidt 2006), ineffective feeding on structural components of labeled litter (i.e., leaf veins and petioles; Suárez et al. 2006a; Filley et al. 2008), and earthworm biomass loss over the course of the experiment. Levels of litter-derived 13C recovery were similar to those reported in field-based tracer studies of litter-derived C redistribution in a northern hardwood forest by Fahey et al. (2012), where 0.61 % of excess 13C lost from labeled sugar maple litter was recovered in earthworm biomass (Lumbricus spp., Aporrectodea spp., and Octolasion tyrtaeum) after 190 days. Litter-derived 13C isotope enrichment in litter-feeding Lumbricus spp. was also significantly greater than that observed in endogeic species (Fahey et al. 2012). Increased litter incorporation into biomass of leaf litter-feeding earthworm species, and increased nutrient assimilation in total earthworm biomass through intraspecific interactions, appears to be a consistent result across ecologically diverse earthworm communities (Cortez et al. 1989; Zhang and Hendrix 1995; Fahey et al. 2013).
Litter-derived C:N ratios in anecic species biomass indicated higher assimilation of leaf litter C than N, and increased assimilation of litter-derived N in All Species treatments of both soil types. Although overall C:N ratios of endogeic species biomass were slightly lower than for anecic and epigeic species, these differences were not significant and may point to some degree of homeostasis in the stoichiometric composition of earthworm tissues across species and soil types (Marichal et al. 2011). Litter-derived C:N ratios in epigeic and endogeic species biomass also indicated higher assimilation of leaf litter C than N, but lower assimilation of litter-derived N than that of litter-feeding anecic species. Earthworm assimilation efficiencies vary greatly with the quality of organic matter ingested, but as shown here and in previous studies, efficiencies are generally higher in litter-feeding earthworm species despite higher rates of organic matter consumption by endogeic species (Whalen and Parmelee 1999; Curry and Schmidt 2006).
Acer rubrum-derived C and N transport and loss
We observed clear differences in litter-derived C and N redistribution across earthworm community treatments and soil textures. First, C losses as CO2 and DOC derived from A. rubrum litter accounted for the largest portion of A. rubrum C removed from surface litter. Second, the A-horizon and burrows were principal sinks for A. rubrum C and N that was transported into the soil, with increased transport and decreased losses associated with multi-species earthworm community activity. Finally, A. rubrum N was primarily redistributed and retained within the soil matrix with relatively small gaseous and leachate losses compared to those of A. rubrum C. Differences in the relative retention and loss of A. rubrum C and N were reflected in lower litter-derived C:N recovery ratios in A-horizon and burrow soils directly altered by earthworm activity.
Our findings are consistent with observations of decreased C:N ratios in surface soils altered by exotic earthworm activity in northern temperate forest soils (Bohlen et al. 2004b; Reich et al. 2005; Hale et al. 2005b; Eisenhauer et al. 2007), which have been attributed to the selective removal of leaf litter of lower C:N ratios from the soil surface and mixing of soil horizons due to feeding and burrowing activity. Narrowing C:N ratios are in turn indicative of shifts toward faster cycling systems with disproportionate effects on net losses of C from northern temperate forest soils (Bohlen et al. 2004b) following earthworm introductions.
Earthworm burrow system structure and soil chemistry associations
Patterns of burrow system continuity, size distribution, and volume were in agreement with the known behavior of the different ecological groups (Bastardie et al. 2005); surface-soil burrow system properties were most associated with endogeic and epigeic species, and sub-soil burrow system properties are most associated with anecic species. Importantly, quantification of burrow system structures and associations with measures of organic matter redistribution indicated two distinct functions of surface-soil and sub-soil burrow system properties. Sub-soil burrow systems with high levels of continuity and large burrow size produced by vertical-burrowing and litter-feeding of anecic species were associated with leaf litter translocation and redistribution into sub-soils, in addition to leachate losses of organic C from the temperate forest soils studied here. Surface burrow systems with the highest volume, greatest surface connectivity, and dense burrow networks in the A-horizon were a product of interspecific interactions between epigeic species and endogeic species. These properties were, in turn, associated with greater CO2 losses and greater transport of leaf litter C and N into the A-horizon.
Increased C losses associated with greater soil porosity observed in this study contrast with results from a previous experiment in which burrow systems of earthworm communities and C losses were assessed over one year, and no correlations between soil porosity shifts due to earthworm activity and CO2 and DOC losses were detected (Crumsey et al. 2013a). The present study demonstrates the short-term (i.e., months following the onset of earthworm activity) biotic control of exotic earthworm communities on soil C losses driven by burrow system production (which likely increases rates of CO2 diffusion and dissolved C infiltration), enhanced leaf litter processing, and organic matter redistribution. In contrast, our previous study indicated that longer-term CO2 and DOC losses, observed over the course of 1 year, may be controlled by heterotrophic respiration rates in the soil matrix rather than CO2 diffusion rates or rates of dissolved C infiltration in temperate forest soils.
Conclusions
Our results demonstrate how substantial earthworm community effects on C and N dynamics and soil structure are influenced by soil texture, and how community and texture factors interact to determine leaf litter transport, loss, and its potential retention in temperate forest soils. The fates of litter-derived C and N in this study likely reflect those of short-term responses of forest soils to earthworm introductions, following the initial production of sub-surface burrow systems, enhanced leaf litter processing, and organic matter redistribution. At these short time scales, the strong influence of soil texture on litter-derived C retention and feedbacks with earthworm-community controls on litter-derived C and N redistribution will likely propagate to differences in long-term trajectories of soil C dynamics across temperate forest soils. Future long-term studies that account for earthworm community dynamics, other drivers of soil C and N dynamics (e.g., belowground effects of tree species, leaf litter loading, and the broader soil faunal community), and are accompanied by direct measures of organic matter stabilization, will enhance our ability to determine the consequences of different earthworm-altered soil C storage trajectories in northern temperate forests.
References
Alban DH, Berry EC (1994) Effects of earthworm invasion on morphology, carbon, and nitrogen of a forest soil. Appl Soil Ecol 1(3):243–249
Bastardie F, Capowiez Y, Cluzeau D (2005) 3D characterisation of earthworm burrow systems in natural soil cores collected from a 12-year-old pasture. Appl Soil Ecol 30(1):34–46
Bird JA, Torn MS (2006) Fine roots vs. needles: a comparison of 13C and 15N dynamics in a ponderosa pine forest soil. Biogeochemistry 79(3):361–382
Bird JA, Kleber M, Torn MS (2008) 13C and 15N stabilization dynamics in soil organic matter fractions during needle and fine root decomposition. Org Geochem 39(4):465–477
Bohlen PJ, Groffman PM, Fahey TJ, Fisk MC, Suárez E, Pelletier DM, Fahey RT (2004a) Ecosystem consequences of exotic earthworm invasion of north temperate forests. Ecosystems 7(1):1–12
Bohlen PJ, Pelletier DM, Groffman PM, Fahey TJ, Fisk MC (2004b) Influence of earthworm invasion on redistribution and retention of soil carbon and nitrogen in northern temperate forests. Ecosystems 7(1):13–27
Brown GG, Barois I, Lavelle P (2000) Regulation of soil organic matter dynamics and microbial activity in the drilosphere and the role of interactions with other edaphic functional domains. Eur J Soil Biol 36(3):177–198
Burtelow AE, Bohlen PJ, Groffman PM (1998) Influence of exotic earthworm invasion on soil organic matter, microbial biomass and denitrification potential in forest soils of the northeastern United States. Appl Soil Ecol 9(1):197–202
Capowiez Y, Pierret A, Daniel O, Monestiez P, Kretzschmar A (1998) 3D skeleton reconstructions of natural earthworm burrow systems using CAT scan images of soil cores. Biol Fertil Soils 27(1):51–59
Capowiez Y, Sammartino S, Michel E (2011) Using X-ray tomography to quantify earthworm bioturbation non-destructively in repacked soil cores. Geoderma 162(1):124–131
Cortez J, Hameed R, Bouche MB (1989) C and N transfer in soil with or without earthworms fed with 14C-and 15N-labelled wheat straw. Soil Biol Biochem 21(4):491–497
Costello DM, Lamberti GA (2009) Biological and physical effects of non-native earthworms on nitrogen cycling in riparian soils. Soil Biol Biochem 41(10):2230–2235
Crumsey JM, Le Moine JM, Capowiez Y, Goodsitt MM, Larson SC, Kling GW, Nadelhoffer KJ (2013a) Community-specific impacts of exotic earthworm invasions on soil carbon dynamics in a sandy temperate forest. Ecology 94(12):2827–2837
Crumsey JM, Le Moine JM, Vogel CS, Nadelhoffer KJ (2013b) Historical patterns of exotic earthworm distributions inform contemporary associations with soil physical and chemical factors across a northern temperate forest. Soil Biol Biochem 68:503–514
Curry JP, Schmidt O (2006) The feeding ecology of earthworms—a review. Pedobiologia 50(6):463–477
Curtis PS, Vogel CS, Gough CM, Schmid HP, Su HB, Bovard BD (2005) Respiratory carbon losses and the carbon-use efficiency of a northern hardwood forest, 1999-2003. New Phytol 167(2):437–455
Dray S, Dufour AB (2007) The ade4 package: implementing the duality diagram for ecologists. J Stat Softw 22(4):1–20
Dray S, Chessel D, Thioulouse J (2003) Co-inertia analysis and the linking of ecological data tables. Ecology 84(11):3078–3089
Eisenhauer N, Partsch S, Parkinson D, Scheu S (2007) Invasion of a deciduous forest by earthworms: changes in soil chemistry, microflora, microarthropods and vegetation. Soil Biol Biochem 39(5):1099–1110
Fahey TJ, Yavitt JB, Sherman RE, Groffman PM, Fisk MC, Maerz JC (2011) Transport of carbon and nitrogen between litter and soil organic matter in a northern hardwood forest. Ecosystems 14(2):326–340
Fahey TJ, Yavitt JB, Sherman RE, Maerz JC, Groffman PM, Fisk MC, Bohlen PJ (2012) Earthworms, litter and soil carbon in a northern hardwood forest. Biogeochemistry 114(1–3):269–280
Fahey TJ, Yavitt JB, Sherman RE, Maerz JC, Groffman PM, Fisk MC, Bohlen PJ (2013) Earthworm effects on the incorporation of litter C and N into soil organic matter in a sugar maple forest. Ecol Appl 23(5):1185–1201
Filley TR, McCormick MK, Crow SE, Szlavecz KE, Whigham DF, Johnston CT, van den Heuvel R (2008) Comparison of the chemical alteration trajectory of Liriodendron tulipifera L. leaf litter among forests with different earthworm abundance. J Geophys Res 113.G1
Fisk MC, Fahey TJ, Groffman PM, Bohlen PJ (2004) Earthworm invasion, fine-root distributions, and soil respiration in north temperate forests. Ecosystems 7(1):55–62
Garten CT, Hanson PJ, Todd DE, Lu BB, Brice DJ (2008) Natural 15N- and 13C-abundance as indicators of forest nitrogen status and soil carbon dynamics. In: Michener RH, Lajtha K (eds) Stable isotopes in ecology and environmental science. Blackwell Publishing, Oxford, pp 61–82
Gaudinski JB, Trumbore SE, Davidson EA, Zheng S (2000) Soil carbon cycling in a temperate forest: radiocarbon-based estimates of residence times, sequestration rates and partitioning of fluxes. Biogeochemistry 51(1):33–69
Gough CM, Vogel CS, Schmid HP, Curtis PS (2008) Controls on annual forest C storage: lessons from the past and predictions for the future. Bioscience 58(7):609–622
Hale CM, Frelich LE, Reich PB (2005a) Exotic European earthworm invasion dynamics in northern hardwood forests of Minnesota, USA. Ecol Appl 15(3):848–860
Hale CM, Frelich LE, Reich PB, Pastor J (2005b) Effects of European earthworm invasion on soil characteristics in northern hardwood forests of Minnesota, USA. Ecosystems 8(8):911–927
Harrell FE (2012) Hmisc: Harrell Miscellaneous Package. R package version 3.10-1. http://CRAN.R-project.org/package=Hmisc
Hayes JM (2004) An introduction to isotopic calculations. Woods Hole Oceanographic Institute, Woods Hole
Hendriksen NB (1990) Leaf litter selection by detritivore and geophagous earthworms. Biol Fertil Soils 10(1):17–21
Holdsworth AR, Frelich LE, Reich PB (2007) Regional extent of an ecosystem engineer: earthworm invasion in northern hardwood forests. Ecol Appl 17(6):1666–1677
Holdsworth AR, Frelich LE, Reich PB (2008) Litter decomposition in earthworm-invaded northern hardwood forests: role of invasion degree and litter chemistry. Ecoscience 15(4):536–544
Hughes MS, Bull CM, Doube BM (1996) Microcosm investigations into the influence of sheep manure on the behaviour of the geophagous earthworms Aporrectodea trapezoides and Microscolex dubuis. Biol Fertil Soils 22(1–2):71–75
Jégou D, Cluzeau D, Wolf HJ, Gandon Y, Trehen P (1997) Assessment of the burrow system of Lumbricus terrestris, Aporrectodea giardi, and Aporrectodea caliginosa using X-ray computed tomography. Biol Fertil Soils 26(2):116–121
Jégou D, Cluzeau D, Hallaire V, Balesdent J, Tréhen P (2000) Burrowing activity of the earthworms Lumbricus terrestris and Aporrectodea giardi and consequences on C transfers in soil. Eur J Soil Biol 36(1):27–34
Lenth RV, Hervà M (2015) lsmeans: Least-Squares Means. R package version 2.16. http://CRAN.R-project.org/package=lsmeans
Li XY, Fisk MC, Fahey TJ, Bohlen PJ (2002) Influence of earthworm invasion on soil microbial biomass and activity in a northern hardwood forest. Soil Biol Biochem 34(12):1929–1937
Lussenhop J, Fogel R, Pregitzer K (1991) A new dawn for soil biology—video analysis of root soil microbial faunal interactions. Agric Ecosyst Environ 34(1):235–249
Marhan S, Scheu S (2006) Mixing of different mineral soil layers by endogeic earthworms affects carbon and nitrogen mineralization. Biol Fertil Soils 42(4):308–314
Marichal R, Mathieu J, Couteaux M-M, Mora P, Roy J, Lavelle P (2011) Earthworm and microbe response to litter and soils of tropical forest plantations with contrasting C: N: P stoichiometric ratios. Soil Biol Biochem 43(7):1528–1535
McInerney M, Bolger T (2000) Temperature, wetting cycles and soil texture effects on carbon and nitrogen dynamics in stabilized earthworm casts. Soil Biol Biochem 32(3):335–349
McLean MA, Parkinson D (1997) Soil impacts of the epigeic earthworm Dendrobaena octaedra on organic matter and microbial activity in lodgepole pine forest. Can J For Res 27(12):1907–1913
Nadelhoffer KJ, Fry B (1988) Controls on natural nitrogen-15 and carbon-13 abundances in forest soil organic matter. Soil Sci Soc Am J 52(6):1633–1640
Nadelhoffer KJ, Fry B (1994) Nitrogen isotope studies in forest ecosystems. In: Lajtha K, Michener H (eds) Stable isotopes in ecology and environmental science. Blackwell Scientific Publications, Oxford
Nadelhoffer K, Bowden R, Boone R, Lajtha K (1999a) Controls on forest soil organic matter development and dynamics, chronic litter manipulation as a potential international LTER activity. In: Cooperation in long term ecological research in central and eastern Europe, proceedings of the ILTER regional workshop, pp. 22–25
Nadelhoffer KJ, Downs MR, Fry B (1999b) Sinks for 15N-enriched additions to an oak forest and a red pine plantation. Ecol Appl 9(1):72–86
National Resources Conservation Service (1991) Soil survey of Cheboygan County, Michigan. United States Department of Agriculture, Washington D.C
Nave LE, Gough CM, Maurer KD, Bohrer G, Hardiman BS, Le Moine J, Munoz AB, Nadelhoffer KJ, Sparks JP, Strahm BD, Vogel CS, Curtis PS (2011) Disturbance and the resilience of coupled carbon and nitrogen cycling in a north temperate forest. J Geophys Res Biogeosci 116:G4
Pierret A, Capowiez Y, Belzunces L, Moran CJ (2002) 3D reconstruction and quantification of macropores using X-ray computed tomography and image analysis. Geoderma 106(3):247–271
Pinheiro J, Bates D, DebRoy S, Sarkar D, R Core Team (2015) nlme: Linear and nonlinear mixed effects models
R Development Core Team. 2013. R: a language and environment for statistical computing. Vienna
Reich PB, Oleksyn J, Modrzynski J, Hobbie SE, Eissenstat DM, Chorover J, Chadwick OA, Hale CM, Tjoelker MG (2005) Linking litter calcium, earthworms and soil properties: a common garden test with 14 tree species. Ecol Lett 8(8):811–818
Resner K, Yoo K, Sebestyen SD, Aufdenkampe A, Hale C, Lyttle A, Blum A (2014) Invasive earthworms deplete key soil inorganic nutrients (Ca, Mg, K, and P) in a northern hardwood forest. Ecosystems 18(1):89–102
Scheu S, Parkinson D (1994) Effects of earthworms on nutrient dynamics, carbon turnover and microorganisms in soils from cool temperate forests of the Canadian Rocky Mountains—laboratory studies. Appl Soil Ecol 1(2):113–125
Sollins P, Homann P, Caldwell BA (1996) Stabilization and destabilization of soil organic matter: mechanisms and controls. Geoderma 74(1):65–105
Straube D, Johnson EA, Parkinson D, Scheu S, Eisenhauer N (2009) Nonlinearity of effects of invasive ecosystem engineers on abiotic soil properties and soil biota. Oikos 118(6):885–896
Suárez ER, Fahey TJ, Yavitt JB, Groffman PM, Bohlen PJ (2006a) Patterns of litter disappearance in a northern hardwood forest invaded by exotic earthworms. Ecol Appl 16(1):154–165
Suárez ER, Tierney GL, Fahey TJ, Fahey R (2006b) Exploring patterns of exotic earthworm distribution in a temperate hardwood forest in south-central New York, USA. Landsc Ecol 21(2):297–306
Swanston CW, Torn MS, Hanson PJ, Southon JR, Garten CT, Hanlon EM, Ganio L (2005) Initial characterization of processes of soil carbon stabilization using forest stand-level radiocarbon enrichment. Geoderma 128(1):52–62
Tiunov AV, Hale CM, Holdsworth AR, Vsevolodova-Perel TS (2006) Invasion patterns of Lumbricidae into the previously earthworm-free areas of northeastern Europe and the western Great Lakes region of North America. Biol Invasions 8(6):1223–1234
Torn MS, Trumbore SE, Chadwick OA, Vitousek PM, Hendricks DM (1997) Mineral control of soil organic carbon storage and turnover. Nature 389(6647):170–173
Waldrop MP, Zak DR, Sinsabaugh RL, Gallo M, Lauber C (2004) Nitrogen deposition modifies soil carbon storage through changes in microbial enzymatic activity. Ecol Appl 14(4):1172–1177
Whalen JK, Parmelee RW (1999) Quantification of nitrogen assimilation efficiencies and their use to estimate organic matter consumption by the earthworms Aporrectodea tuberculata (Eisen) and Lumbricus terrestris L. Appl Soil Ecol 13(3):199–208
Wironen M, Moore TR (2006) Exotic earthworm invasion increases soil carbon and nitrogen in an old-growth forest in southern Quebec. Can J For Res 36(4):845–854
Zak DR, Host GE, Pregitzer KS (1989) Regional variability in nitrogen mineralization, nitrification, and overstory biomass in northern Lower Michigan. Can J For Res 19(12):1521–1526
Zeller B, Colin-Belgrand M, Dambrine E, Martin F (2001) Fate of nitrogen released from 15N-labeled litter in European beech forests. Tree Physiol 21(2–3):153–162
Zhang QL, Hendrix PF (1995) Earthworm (Lumbricus rubellus and Aporrectodea caliginosa) effects on carbon flux in soil. Soil Sci Soc Am J 59(3):816–823
Zhang W, Hendrix PF, Dame LE, Burke RA, Wu J, Neher DA, Li J, Shao Y, Fu S (2013) Earthworms facilitate carbon sequestration through unequal amplification of carbon stabilization compared with mineralization. Nat Commun 4:2576
Acknowledgments
We thank M. Grant, C. Vogel, F. Santos, M. Busch, R. Spray, T. Sutterly, S. Webster, and B. Vande Kopple for field and laboratory assistance. We also thank all anonymous reviewers for constructive feedback on this manuscript. The use of equipment and facilities at the University of Michigan Biological Station, the University of Michigan Department of Radiology, the Queens College School of Earth and Environmental Sciences, and the French National Institute of Agricultural Research are deeply appreciated. The National Science Foundation Doctoral Dissertation Improvement Grant #1110494, and the University of Michigan Department of Ecology and Evolutionary Biology provided financial support for this research.
Author information
Authors and Affiliations
Corresponding author
Additional information
Responsible Editor: Jan Mulder.
Electronic supplementary material
Below is the link to the electronic supplementary material.
Rights and permissions
About this article
Cite this article
Crumsey, J.M., Capowiez, Y., Goodsitt, M.M. et al. Exotic earthworm community composition interacts with soil texture to affect redistribution and retention of litter-derived C and N in northern temperate forest soils. Biogeochemistry 126, 379–395 (2015). https://doi.org/10.1007/s10533-015-0164-6
Received:
Accepted:
Published:
Issue Date:
DOI: https://doi.org/10.1007/s10533-015-0164-6