Abstract
Cistanche deserticola has been found to exert protection against aging and age-related diseases, but mechanisms underlying its longevity effects remain largely unclear. Here, the multicellular model organism Caenorhabditis elegans was employed to identify lifespan extending and protective effects against β-amyloid (Aβ) induced toxicity by echinacoside (ECH), a phenylethanoid glycoside isolated from C. deserticola. Our results showed that ECH extends the mean lifespan of worms and increases their survival under oxidative stress. Levels of intracellular reactive oxygen species and fat accumulation were also significantly suppressed by ECH. Moreover, ECH-mediated lifespan extension was found to be dependent on mev-1, eat-2, daf-2, and daf-16, but not sir-2.1 or hsf-1 genes. Furthermore, ECH triggered DAF-16 nuclear localization and upregulated two of its downstream targets, sod-3 and hsp-16.2. In addition, ECH significantly improved the survival of CL4176 worms in response to proteotoxic stress induced by Aβ protein aggregation. Collectively, these findings suggested that reactive oxygen species scavenging, dietary restriction, and insulin/insulin-like growth factor signaling pathways could be partly involved in ECH-mediated lifespan extension. Thus, ECH may target multiple longevity mechanisms to extend lifespan and have a potency to prevent Alzheimer’s disease progression.
Graphical Abstract

Similar content being viewed by others
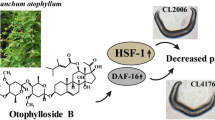
Avoid common mistakes on your manuscript.
Introduction
An increasing aged population is becoming a social and economic problem in many countries. As such, aging research has rapidly gained broad scientific interest. The discovery of new botanical compounds with lifespan-extending effects could prompt new strategies for treating age-related diseases such as diabetes, cancer, and neurodegenerative disorders (Chen et al. 2014; Pan et al. 2012).
Caenorhabditis elegans is a well-established model system for investigating organismal aging and exploring new pharmacological targets because of its relatively short lifespan, rapid life cycle, ease of cultivation, and well-known genetic pathways, which are conserved across diverse species including mammals (Honda et al. 2010). Indeed, a reported 60–80% of human gene homologues have been identified in C. elegans. Thus, it has been increasingly applied to investigate the effects of pharmacologically active compounds on aging processes (Kaletta and Hengartner 2006).
Cistanche deserticola Y. C. Ma (C. deserticola), commonly called Rou Cong Rong in Chinese, is a holoparasitic plant distributed throughout semi-arid to arid areas that has long been used by Chinese and Japanese as a traditional herbal medicine to treat kidney deficiency, impotence, morbid leucorrhea, neurasthenia, and senile constipation (China Pharmacopoeia Committee 2010). Modern phytochemical and pharmacological studies on Cistanche species led to isolation and identification of various components including phenylethanoid glycosides (PhGs), lignans, iridoids, and polysaccharides (Jiang and Tu 2009). Importantly, some isolated components displayed hepatoprotective, neuroprotective, anti-aging, anti-nociceptive, anti-inflammatory, anti-bacterial, anti-fatigue, and anti-oxidant effects (Nan et al. 2013; Guo et al. 2016; Lin et al. 2002; Cai et al. 2010; Fu et al. 2008; Wang et al. 2015).
Echinacoside (ECH), a major compound of PhGs in C. deserticola, has exhibited multiple bioactivities, such as anti-aging, anti-oxidation, anti-inflammatory, hepatoprotective, and neuroprotective effects (Cai et al. 2010; Fu et al. 2008). One study showed that ECH prolonged longevity in C. elegans through the daf-16 transcription factor (Wang et al. 2015). However, molecular mechanisms underlying lifespan extension and health benefits of ECH in this whole animal model are still under discussion.
Here, we extensively investigated molecular mechanisms of ECH-mediated longevity using transgenic C. elegans. We found that ECH improved the survival of C. elegans in response to proteotoxic stress induced by Aβ aggregation. Thus, our findings support the possibility of natural chemical interventions for aging and aging-related processes.
Materials and methods
Extraction and isolation of PhGs
Caenorhabditis deserticola plant material purchased from Kangqiao Pharmaceutical Co. Ltd. (Shanghai, China) was extracted (200 g) in 70% ethanol for 2 h using a reflux method. This process was repeated twice before combining extracts and concentrating using vacuum-rotary evaporation. Subsequently, extracts were suspended in water and partitioned using ethyl acetate and n-butanol successively. N-butanol partitioned fractions were combined and concentrated before being suspended in water. The macroporous resin was used for PhG adsorption and separation. PhGs were eluted with 70% ethanol, combined, and evaporated.
Reagents and preparation
Unless otherwise stated, all chemicals were purchased from Sigma-Aldrich (Shanghai, China). ECH (purity of 96.5%, see supplementary data), acteoside (ACT), and isoacteoside (ISO) were purchased from the National Institute for the Control of Pharmaceutical and Biological Products. PhGs, ECH, ACT, and ISO were all dissolved in dimethyl sulfoxide (DMSO). A final DMSO concentration of 0.3% (v/v) was maintained under all conditions.
Strains and maintenance
N2 Bristol (wild-type) C. elegans and transgenic strains CL4176 [(pAF29) myo-3p::Aβ 1-42 + (pRF4) rol-6 (su1006)], daf-2 (e1370), daf16 (mu86), TJ356 [zIs356 IV (daf-16p::daf-16a/b::GFP + rol-6 (su1006)]; PS3551, hsf-1 (sy441); AM140 [rmls132 (unc-54p::Q35::YFP)]; TK22, mev-1 (kn-1), CF1553 [(pAD76) sod-3::GFP + rol6 (su1006)]; eat-2 (ad1116); VC199, sir-2.1 (ok434); CL2070 [hsp-16.2p::GFP + rol-6 (su1006)]; and Escherichia coli (E. coli) OP50 were originally provided by Caenorhabditis Genetics Center (St. Paul, MN). Worms were maintained on nematode growth medium (NGM) plates seeded with E. coli OP50. All worms were cultured at 20 °C unless otherwise stated (Brenner 1974). Synchronized worms were obtained using sodium hypochlorite treatment (Fabian and Johnson 1994).
Lifespan assay
Synchronized worms were transferred to 96-well plates containing liquid NGM with E. coli OP50. Progeny development was blocked at the L4 stage using 200-µM 5-fluoro-2′-deoxyuridine (FUDR). Adult worms were treated with PhGs or DMSO (0.3%) control at day 0; thus, treatment day was considered as day 0 of their lifespan. Worms were then observed and scored every 2 days for survival. To avoid starvation, E. coli OP50 was added during assays.
Phenotype analysis
Synchronized N2 worms were placed on E. coli OP50-seeded NGM plates with or without various concentrations of ECH (0, 2, 20, and 200 µM). For measurements of body movement or pharynx pumping rate, 200-µM FUDR was added to block progeny development at the L4 stage. Body movement of adult worms at day 0 and day 2 was measured by scoring the number of body bends in a 20-second interval using a dissecting microscope. Number of adult worms’ pharyngeal contractions was also counted for 20 s at day 0 and day 5 to assess pharynx pumping rate. Both body movement and pharynx pumping rate assays were repeated thrice with 30 worms per assay. For the reproduction assay, L4 worms were individually transferred to a fresh plate containing ECH or DMSO control each day until reproduction ceased. The number of offspring from a single worm was counted at their L2 or L3 stage. Each experiment assessed 30 worms.
Stress resistance
For the thermal-tolerance assay, synchronized N2 worms were placed on E. coli OP50-seeded NGM plates with or without ECH. Two-hundred µM FUDR was added to block progeny development at the L4 stage. Adult worms at day 0 were incubated at 37 °C until all worms had died. Survival of worms was scored every hour and determined using the touch-provoke method. Worms were scored as dead when they failed to respond to touch using a platinum wire pick.
To assess resistance to oxidative stress, synchronized N2 worms were transferred to 96-well plates containing liquid NGM with E. coli OP50. Simultaneously, L1 worms were treated with or without ECH. FUDR (200 µM) was added to block progeny development at the L4 stage. Adults were collected and washed twice using S-complete medium. Worms were then suspended in S-complete before being transferred to a fresh 96-well plate. Ten mM hydrogen peroxide (H2O2) was added and the number of dead worms was scored every hour until all worms had died.
Measurement of intracellular reactive oxygen species (ROS)
Synchronized N2 worms or mev-1 mutants were grown in liquid NGM with E. coli OP50 and treated with or without ECH. FUDR (200 µM) was added to block progeny development at the L4 stage. Adult worms at day 5 were treated with 100-mM 2,7-dichlorodihydrofluorescein diacetate (H2DCF-DA) and incubated at 37 °C for 2 h. Fluorescence was then measured at 485-nm excitation and 530-nm emission. Each assay was repeated thrice with 20 worms per assay.
Nile red staining
Synchronized N2 worms were transferred to 96-well plates and raised in liquid NGM with E. coli OP50. Worms were treated with or without 200 µM ECH. FUDR (200 µM) was added to block progeny development at the L4 stage. On day 3 of adulthood, wells were supplemented with Nile red (75 ng/mL) for 2 days. Worms were then collected and washed twice using S-complete medium before being transferred to a fresh plate. Worms were fixed on a microscope slide using paraformaldehyde (4%, v/v) and visualized using fluorescence microscopy. Each experiment assessed 30 worms. Fluorescence intensity was quantified using ImageJ software.
Intracellular localization of DAF-16
Synchronized worms of the TJ356 (daf-16::GFP)transgenic strain were raised in liquid NGM containing E. coli OP50, and treated with or without ECH at the L1 stage. Adult worms at day 0 were collected and washed twice with S-complete medium before being transferred to a fresh plate. Subsequently, worms were fixed on a microscope slide using paraformaldehyde (4%). Cellular localization of DAF-16::GFP was visualized using fluorescence microscopy. Each experiment assessed 30 worms. Images were analyzed using ImageJ software for GFP quantification.
Quantification of gene expression by quantitative real-time PCR
Synchronized N2 worms were raised as described for the oxidative stress assay. Total RNA was extracted from adult worms at day 5 using Trizol reagent. cDNA was produced from 0.5 µg of total RNA per reaction using a real-time PCR kit (TianGen Biotech, Beijing, China). mRNA expression was assessed for sod-3 and hsp-16.2 in comparison with β-actin using the following primers: sod-3, CGAGCTCGAACCTGTAATCAGCCATG (F) and GGGGTACCGCTGATATTCTTCCAGTTG (R); hsp-16.2, CTGCAGAATCTCTCCATCTGAGTC (F) and AGATTCGAAGCAACTGCACC (R); β-actin, GTGTGACGACGAGGTTGCCGCTCTTGTTGTAGAC (F) and GGTAAGGATCTTCATGAGGTAATCAGTAACATCAC (R). Data were analyzed using the 2−ΔΔCT method. Approximately 1000 worms were used for each experiment.
Fluorescence measurement of fluorescent protein-reporter strains
CL2070 (hsp-16.2p::GFP) and CF1553 (sod-3::gfp) transgenic strains were used to detect expression of sod-3 and hsp-16.2,respectively. AM140 (Q35::YFP) was used to examine accumulation of YFP-tagged Q35. Synchronized worms were grown in liquid NGM with OP50, and treated with or without ECH. FUDR (200 µM) was added to block progeny development at the L4 stage. After a washing step with S-complete, adults were placed on a microscope slide and fixed using paraformaldehyde (4%). Worms (30 per experiment) were observed using a fluorescence microscope. Fluorescence intensity was analyzed using ImageJ software.
Chemotaxis assay
Synchronized N2 worms raised in liquid NGM with E. coli OP50 were treated with ECH at the L1 stage. FUDR (200 µM) was added to block progeny development at the L4 stage. On day 5 of adulthood, worms were collected and washed twice with S-complete. Worms were then placed in a defined center of the agar plate containing 10 µL of 1 M sodium acetate on one side and 10 µL of water on the other side. Sodium acetate served as an attractant. Sodium azide was added to paralyze the worms for counting. Numbers of worms at attractant (a) and control (b) locations were counted after 90 min. By taking the difference of a and b and dividing by the total number of worms (a + b), the chemotaxis index (CI) was calculated as follows: CI = (a − b)/(a + b) (Matsuura et al. 2004).
Worm paralysis assay
Synchronized CL4176 worms were placed on NGM plates containing E. coli OP50. Worms were treated at the L1 stage with 200 µM ECH or 25 µM ginkgolide A as positive controls (Wu et al. 2006). Following incubation at 16 °C for 36 h, the culture temperature was adjusted up to 23 °C to induce expression of Aβ. After 24 h, worms were observed and scored as paralyzed if they failed to undergo full body wave propagation in response to touching with a platinum pick.2.14 Statistical analysis.
All assays were performed independently two or three times. Statistical analysis was performed using GraphPad Prism 6 software. Lifespan, stress resistance, and paralysis assays were analyzed using the Kaplan–Meier survival method. Significant differences were determined using a log-rank test. For other assays, P values were determined using a t test. Differences were considered significant at P < 0.05.
Results
Total PhGs and ECH increased mean lifespan of C. elegans
To investigate whether total PhGs (ECH accounted for 43.8% of extract, Fig. S2) isolated from C. deserticola extended the lifespan of wild-type C. elegans under normal conditions, adult worms were exposed to total PhGs (100 or 500 µg/mL) at day 0 and observed every 2 days for survival. As shown in Fig. 1a and Table 1, the higher concentration tested extended mean lifespan by 15.98% compared with DMSO control, whereas the lower concentration hardly extended mean lifespan. Previous studies have shown that ECH, ACT, and ISO are the major PhGs in C. deserticola (Li et al. 2008). To evaluate whether these three compounds affected the lifespan of C. elegans, wild-type worms were treated with ECH, ACT, or ISO at concentrations of 0, 2, 20, and 200 µM. We found that worms treated with 200 µM ECH not only exhibited significant changes in the survival curve, but also produced a 13.64% increase in mean lifespan (Fig. 1b; Table 1). In contrast, neither ACT nor ISO modulated nematode longevity (Fig. S1, Table 1), indicating that ECH was responsible for the longevity-prolonging effect of PhGs in C. elegans.
Total PhGs and ECH increase the lifespan of C. elegans. Synchronized N2 worms were raised in liquid NGM containing E. coli OP50. FUDR (200 μM) was added at the L4 stage. Following exposure to various concentrations of PhGs, the adults were scored every 2 days for survival. a 500 μg/mL, but not 100 μg/mL, of the total PhGs significantly extended the mean lifespan of the worms by 15.98% in comparison with DMSO controls. Control, n = 165; PhGs (100 μg/mL), n = 185; PhGs (500 μg/mL), n = 204, **P < 0.01. b ECH was able to prolong the longevity of wild-type C. elegans in a dose-dependent manner from 2 to 200 μM. ECH (200 μM) significantly increased the mean lifespan by 13.64% compared with DMSO controls. Control, n = 123; ECH (200 μM), n = 130. *P < 0.05
ECH does not affect age-related physiological functions of C. elegans
To examine whether ECH affected the physiology of C. elegans, we analyzed changes in three age-related physiological functions in worms treated with ECH at concentrations of 0, 2, 20, or 200 µM. First, we investigated whether ECH treatment affected the body movement of worms on day 0 and day 2. Our findings suggested that ECH treatment did not affect nematode body movement at these times (Fig. 2a, b; Table S1). To evaluate the effect of ECH on pharyngeal pumping, the rate of pharyngeal contraction of worms treated with or without ECH was scored on days 0 and day 5. ECH treatment at various doses did not significantly alter pharyngeal pumping rates at these times (Fig. 2c, d; Table S2). Finally, we recorded the effect of ECH treatment on offspring production to explore whether ECH suppressed this process. As shown in Fig. 2e, no significant differences in offspring production were observed between ECH-treated and control groups, indicating that ECH did not influence the production of progeny by C. elegans (Fig. 2e; Table S3). To summarize, ECH treatment prolonged the mean lifespan of worms without causing obvious defects in body movement, pharyngeal pumping, or reproductive capacity.
Effect of ECH on age-related physiological functions. Synchronized N2 worms grown on E. coli OP50 seeded NGM plates were treated with various concentrations of ECH (0, 2, 20, 200 μM). a and b The body movement of adult day 0 and day 2 worms was measured by scoring the number of body bends in an interval of 20 s. ECH could not significantly alleviate the decline in body movement. c and d The pharynx pumping rate of adult day 0 and day 5 worms was scored in an interval of 20 s. ECH could not significantly rescue the decline in pharyngeal pumping on day 5 of adulthood compared with day 0 worms. e The number of offspring from a single worm was counted at their L2 or L3 stage. ECH treatment did not significantly impact the number of offspring in C. elegans
ECH enhances the resistance of worms to heat and oxidative stress
Increased longevity is closely associated with improved the survival of C. elegans under conditions of heat or oxidative stress (Larsen 1993). To determine whether ECH enhanced thermal tolerance, wild-type worms pretreated with 200 µM ECH were exposed to heat stress at 37 °C. Survival curves showed that C. elegans were sensitive to thermal stress while ECH treatment could alleviate this lethality. Compared with the control, the survival curve of the ECH-treated group showed a significant shift to the right. Specifically, 200 µM ECH extended maximum lifespan from 7 to 11 h, and significantly prolonged mean lifespan by 40.20% under thermal stress (Fig. 3a; Table S4). To investigate whether ECH exerts oxidative stress resistance properties, wild-type worms were exposed to H2O2 (10 mM), an intracellular ROS inducer. Similarly, the ECH-treated group presented an observably different survival curve and 10.97% increase in mean lifespan under H2O2-induced oxidative stress in the presence of 200 µM ECH (Fig. 3b; Table S5). These findings indicated that ECH enhanced resistance to both thermal and oxidative stresses.
ECH enhances resistance to heat and oxidative stress. a Synchronized N2 worms were placed on E. coli OP50 seeded NGM plates with or without ECH. On day 0 of adulthood, worms were incubated at 37 °C until all of them had died. Survival of the worms was scored every hour. ECH at 200 μM significantly increased the mean lifespan of C. elegans by 40.20% under thermal stress at 37 °C compared with DMSO controls. Control, n = 155; ECH (200 μM), n = 166. ***P < 0.001. b Synchronized N2 worms were transferred into liquid NGM with E. coli OP50. Simultaneously, the L1 worms were supplemented with or without ECH. On day 0 of adulthood, worms were treated with H2O2 (10 mM). The worms were scored every hour until all the worms had died. ECH at 200 μM observably increased the mean lifespan of C. elegans by 10.97% under H2O2-induced oxidative stress in comparison with untreated controls. Control, n = 224; ECH (200 μM), n = 173. ***P < 0.001
ECH reduced intracellular ROS andfat accumulation
Both oxidative and heat stresses can cause cellular damage through ROS accumulation, and ROS overproduction is a prominent feature of the aging process (Finkel and Holbrook 2000). We suspected that ECH may induce longevity through suppressing ROS levels. Therefore, we examined the ROS-scavenging ability of ECH. ROS was measured in wild-type worms using an H2DCF-DA fluorescent probe, which is able to cross cell membranes before being converted to fluorescent DCF by ROS. The results showed that ECH supplementation (200 µM) dramatically lowered intracellular ROS levels to 59.00% compared with untreated controls (Fig. 4a; Table S6).
ECH reduces ROS levels and fat accumulation. Synchronized N2 worms raised in liquid NGM were treated with or without ECH at the L1 stage. a Adult day 5 worms were treated with 100 mM H2DCF-DA for measurement of intracellular ROS levels. ECH at 200 μM significantly reduced ROS levels to 59.00%. ***P < 0.001. b Adult day 5 worms were stained using Nile red (75 ng/mL) for 2 days. The worms were visualized using fluorescence microscopy. ECH at 200 μM markedly reduced fat accumulation to 79.50%. ***P < 0.001
To assess the effect of ECH on fat accumulation, wild-type N2 worms pretreated with or without ECH were stained with Nile red. Fluorescence intensity measurements revealed that 200 µM ECH was able to significantly reduce fat accumulation to 79.50% of untreated controls (Fig. 4b; Table S7).
ECH-mediated oxidative stress resistance is dependent on mev-1
To further investigate the oxidative stress-resistance properties of ECH, we examined the effect of ECH on TK22, mev-1 (kn-1) worms harboring a mutation in the cytochrome b large subunit of mitochondrial complex II. This mutation leads to overproduction of superoxide, which increases oxidative stress and premature aging, and reduces lifespan (Ishii et al. 1998). The results showed no increase in the lifespan of mev-1 (kn-1) worms treated with or without 200 µM ECH (Fig. 5; Table 1). Moreover, the ROS-scavenging ability of ECH was weakened in mev-1 mutants (Fig. S4), suggesting that the protective effect of ECH against oxidative stress requires the mev-1 gene, which is important for endogenous detoxification pathways.
mev-1 is required for the lifespan extension produced by ECH. Synchronized mev-1 (kn-1) mutant worms were raised in liquid NGM containing E. coli OP50. FUDR (200 μM) was added at the L4 stage. Following exposure to ECH (200 μM), the worms were scored every 2 days for survival. ECH failed to increase the lifespan of mev-1 mutants. Control, n = 151; ECH (200 μM), n = 129
ECH extends lifespan through the dietary restriction pathway
Dietary restriction (DR) is a well-known mechanism of lifespan extension that has been well established in C. elegans (Lakowski and Hekimi 1998; Kenyon 2010). To explore whether ECH-mediated lifespan extension occurred through the DR pathway, the effect of ECH on the lifespan of an eat-2 (ad1116) mutant was investigated. This long-lived mutant worm is a genetic model of DR because it causes a reduction in pharyngeal pumping rate and food intake in C. elegans (McKay et al. 2004).
As shown in Fig. 6a and Table 1, treatment with 200 µM ECH suppressed the survival of eat-2 (ad1116) mutants, causing a 10.61% decline in mean lifespan. This indicated that ECH-mediated prolongation of longevity is highly depended on the eat-2 (ad1116) gene. Given one feature of DR in C. elegans is a reduction of pharyngeal pumping, we demonstrated that ECH affected neither pharyngeal pumping rate (Fig. 2c, d; Table S2) nor influenced the food intake of C. elegans (Fig. S3). Taken together, these results suggested that the longevity extension produced by ECH was associated with cellular signaling pathways involved in DR response without altering feeding capacity.
ECH extends lifespan through the DR pathway. Synchronized eat-2 (ad1116) or sir-2.1 (ok434) mutant worms were raised in liquid NGM containing E. coli OP50. FUDR (200 μM) was added at the L4 stage. Following exposure to ECH (200 μM), the worms were scored every 2 days for survival. a ECH at 200 μM reduced the mean lifespan of eat-2 (ad1116) mutants by 10.61%. Control, n = 162; 200 μM ECH, n = 143. *P < 0.05. b ECH at 200 μM increased the mean lifespan of sir-2.1 (ok434) mutants by 16.77%. Control, n = 142; ECH (200 μM), n = 120. *P < 0.05
Sir2 has been observed to regulate DR in yeast, although its knockout failed to prolong longevity (Guarente and Picard 2005). Sir-2.1, which encodes a NAD-dependent protein deacetylase responsible for DR-mediated lifespan extension, was found to be induced upon DR in C. elegans (Bamps et al. 2009). We observed that ECH was still able to significantly prolong longevity in sir-2.1 (ok434) mutants (Fig. 6b; Table 1), indicating that sir-2.1 is not required for the beneficial effects of ECH on lifespan.
ECH extends lifespan through the IIS pathway
The insulin/IGF signaling (IIS) pathway modulates the longevity of many organisms including C. elegans (Altintas et al. 2016). In this pathway, daf-2 and daf-16 are the key targets, with DAF-2 serving as the receptor and daf-16 encoding the DAF-16 transcription factor, an ortholog of mammalian FOXO (Mukhopadhyay et al. 2006).
To determine if ECH extended lifespan by acting through the IIS pathway, we carried out a lifespan assay in daf-2(e1370) and daf-16 (mu86) mutants. Consistently, we found that 200 µM ECH failed to prolong the lifespan of these mutants (Fig. 7a, b; Table 1). The results indicated that ECH-mediated lifespan extension is in part dependent on the IIS pathway.
ECH extends lifespan through the IIS pathway. Synchronized daf-2 (e1370) or daf-16 (mu86) mutant worms were raised in liquid NGM containing E. coli OP50. FUDR (200 μM) was added at the L4 stage. Following exposure to ECH (200 μM), the worms were scored every 2 days for survival. a ECH failed to extend the lifespan of daf-2 (e1370) mutants. P = 0.9077. b ECH failed to extend the lifespan of daf-16 (mu86) mutants. P = 0.7184. c and d Synchronized worms of the TJ356 (daf-16::GFP) transgenic strain raised in liquid NGM containing E. coli OP50 were treated with or without ECH. On day 0 of adulthood, the fluorescence intensity was measured to analyze the cellular distribution of DAF-16::GFP. The nuclear localization of DAF-16.2::GFP was significantly increased from 13.50 to 42.59% by ECH (200 μM). **P < 0.01. e and f Synchronized N2 worms grown in liquid NGM with E. coli OP50 were treated with or without ECH at the L1 stage. FUDR (200 μM) was added to block progeny development at the L4 stage. Total RNA was extracted from adult day 5 worms. cDNA was produced from the RNA. qRT-PCR was performed with β-actin, sod-3 or hsp-16.2 primers. β-actin was performed as endogenous control. The data was analyzed using the 2−ΔΔCT comparison method. ECH at 200 μM dramatically increased the mRNA levels of sod-3 (71.85-fold; **P < 0.01) and hsp-16.2 (88.60-fold; **P < 0.01). g and h Synchronized worms of the CF1553 (sod-3::GFP) and CL2070 (hsp-16.2p::GFP) transgenic strains were bred in liquid NGM with E. coli OP50 and treated with or without ECH. FUDR (200 μM) was added to block progeny development at the L4 stage. On day 2 of adulthood, the fluorescence was detected. ECH enhanced expression of sod-3 (1.22-fold; *P < 0.05) and hsp-16.2 (1.21-fold; *P < 0.05) in protein levels
Considering that DAF-16 is a transcription factor, we investigated whether ECH could promote DAF-16 nuclear localization using the TJ356 transgenic strain carrying a daf-16::GFP fusion construct as a reporter gene, which allows for observation of the subcellular localization of DAF-16. The results showed that 200 µM ECH significantly increased DAF-16 nuclear localization from 13.50 to 42.59% (Fig. 7c, d; Table S8).
Given that ECH triggers DAF-16 nuclear localization, we evaluated the effect of ECH on expression of sod-3 and hsp-16.2, two downstream targets of DAF-16 in C. elegans (Hesp et al. 2015). SOD-3, a superoxide dismutase, is known to be involved in lifespan extension and oxidative stress resistance. Hsp-16.2 encodes a small heat shock protein that plays a role in promoting longevity (Murphy et al. 2003). Quantitative real-time PCR results demonstrated that mRNA levels of sod-3 and hsp-16.2 were remarkably increased by 71.85-fold and 88.60-fold, respectively, in ECH-treated worms compared with untreated controls (Fig. 7e, f; Table S9).
We further investigated whether ECH modulates protein levels of SOD-3 and HSP-16.2 using CF1553 (sod-3::GFP) and CL2070 (hsp-16.2p::GFP) transgenic strains. CF1553 worms express a GFP-tagged SOD-3 protein, while the CL2070 strain contains a construct in which the gene coding for GFP is coupled to the hsp-16 promoter. Adult worms were observed on day 2 using a fluorescence microscope, with fluorescence intensity correlating to expression of sod-3 or hsp-16.2. The results displayed that ECH significantly enhanced protein expression of SOD-3 (1.22-fold) and HSP-16.2 (1.21-fold) compared with untreated controls (Fig. 7g, h; Table S10).
These results highly supported the possibility that lifespan extending effects of ECH are partially conferred by activating downstream targets of daf-16, such as sod-3 and hsp-16.2. Furthermore, enhanced expression of stress-resistance-related genes could also illustrate why ECH could significantly enhance the resistance of worms to thermal and oxidative stress.
Hsf-1 is not required for ECH-mediated lifespan extension
The heat shock factor HSF-1 is known to regulate protein folding and gene expression in response to heat stress, and is also involved in modulation of longevity (Douglas et al. 2015). Heat shock and caloric restriction reportedly exert a synergistic effect on the heat shock response of C. elegans in a sir2.1-dependent manner (Raynes et al. 2012). Additionally, HSF-1 and DAF-16 have been reported to act together to activate expression of downstream genes promoting longevity (Hsu et al. 2003). Accordingly, we asked whether ECH-mediated lifespan extension required HSF-1. To clarify this question, we tested the effect of 200 µM ECH on hsf-1(sy441) mutants. We observed a significant extension of mean lifespan by 19.78% (Fig. 8; Table 1), suggesting ECH-mediated lifespan extension is independent of hsf-1.
hsf-1 is not required for the lifespan extension produced by ECH. Synchronized hsf-1 (sy441) mutant worms were raised in liquid NGM containing E. coli OP50. FUDR (200 μM) was added at the L4 stage. Following exposure to ECH (200 μM), the worms were scored every 2 days for survival. ECH at 200 μM significantly increased the mean lifespan of hsf-1 (sy441) mutants by 19.78%. Control, n = 157; ECH (200 μM), n = 200. *P < 0.05
ECH increases the chemotaxis index (CI) of C. elegans
To gain insight into ECH-mediated protective effects, we first examined whether ECH affected the physiological behavior of C. elegans, which display declines during the aging process (Kenyon 2010). CI, which is a measure of the learning behavior and response sensitivity to an external stimulus (Matsuura et al. 2004; Bargmann et al. 1993), was measured by placing adult worms on a defined center of an agar plate at day 5. On one side of the center was sodium acetate (1 M), which was used as an attractant, while the other side contained water. Numbers of worms on the two sides was counted after 90 min to calculate CI. The value of CI represents the proportion of worms on the side containing sodium acetate minus the proportion of worms on the side of the water, divided by the total number of worms.
We observed a significant increase in CI in the presence of ECH (Fig. 9; Table S11). This suggested that ECH can attenuate the age-dependent decline in physiological behavior.
ECH increases the chemotaxis index of C. elegans. Synchronized N2 worms raised in liquid NGM with E. coli OP50 were treated with ECH at the L1 stage. FUDR (200 μM) was added at the L4 stage. Adult day 5 worms were placed on a defined center of the agar plate containing 10 μL of 1 M sodium acetate on one side and 10 μL of water on the other side. The number of worms at attractant location (a) and the number of worms at control location (b) were counted after 90 min. The chemotaxis index (CI) was calculated as CI = (a − b)/(a + b). The CI value of worms treated with 200 μM ECH (21.50) was higher than that of the untreated controls (− 23.00). **P < 0.01
ECH protects C. elegans from Aβ toxicity
Alzheimer’s disease (AD) is the most common neurodegenerative disorder in the elderly. Progressive deposition of β-amyloid (Aβ) protein and the accumulation of phosphorylated tau protein are responsible for AD (Luo 2006).
To investigate whether ECH could alleviate proteotoxic stress induced by Aβ accumulation in C. elegans, the CL4176 transgenic strain constitutively expressing human Aβ in body wall muscle cells to result in a paralyzed phenotype at 23 °C, was employed in a paralysis assay. Our findings showed that 200 µM ECH or 25 µMginkgolide A elicited a significant right-shift of the survival curves of CL4176 worms (Fig. 10; Table S12), indicating ECH exerts a protective effect against Aβ-induced toxicity in worms (Wu et al. 2006).
ECH protects C. elegans from Aβ toxicity. Synchronized CL4176 worms were placed on NGM plates containing E. coli OP50. The worms were treated with various concentrations of ECH or 25 μM ginkgolide A (GA) as a positive control at the L1 stage. Following incubation at 16 °C for 36 h, the expression of Aβ was induced by adjusting the culture temperature up to 23 °C. After 24 h, the worms were observed and scored for paralysis. The mean lifespan of CL4176 worms were significantly extended 13.80% (*P < 0.05) by 200 μM ECH and 17.04% (**P < 0.01) by 25 μM GA, respectively. Control, n = 144; GA (25 μM); n = 120; ECH (200 μM), n = 156
ECH fails to alleviate proteotoxic stress induced by polyglutamine aggregation
In humans, polyglutamine (PolyQ) aggregation is considered to be the key pathological factor in Huntington’s disease, an adult-onset neurodegenerative disorder (Morimoto 2008). To determine whether ECH reduced PolyQ accumulation, the AM140 (Q35::YFP) transgenic strain expressing 35 glutamine repeats with a YFP tag in body wall muscle cells, was employed. Worms were treated with various concentrations of ECH (0, 2, 20, or 200 µM) at the L1 stage. On day 4 and day 6 of adulthood, expression of Q35::YFP was analyzed using a fluorescence microscope. However, ECH treatments did not diminish the formation of Q35::YFP (Fig. 11; Table S13), indicating ECH could not alleviate the proteotoxic stress induced by PolyQ aggregation.
ECH fails to alleviate the polyglutamine aggregation. Synchronized worms of the AM140 (Q35::YFP) transgenic strain were grown in liquid NGM with E. coli OP50 and treated with or without ECH at the L1 stage. FUDR (200 μM) was added at the L4 stage. The fluorescence intensity was measured on day 4 (a) and day 6 (b) of adulthood. ECH did not affect the expression of Q35::YFP
Discussion
C. deserticola has been found to possess both anti-aging and anti-oxidation activities. However, to our knowledge, previous reports are limited to alcohol extracts or total PhGs ofC. deserticola(Guo et al. 2016; Wat et al. 2016; Zhang et al. 2014; Nan et al. 2016). Here, we determined the active component of C. deserticola to be ECH, which confers potential enhancement of lifespan and stress resistance in C. elegans.
First, we observed that total PhGs extracted from C. deserticola could significantly extend the mean lifespan of wild-type C. elegans. To establish the material foundation of PhGs for lifespan extension, we investigated ECH, ACT, and ISO—three monomeric compounds representing the major PhGs in C. deserticola (Li et al. 2008). Interestingly, we found that ECH, rather than ACT or ISO, extends the lifespan of C. elegans in a dose-dependent manner. Thus, our results indicated that ECH is responsible for the lifespan extending effects of C. deserticola (Fig. 1b and S1). Additionally, results demonstrating that 200 µM ECH increased the mean lifespan of worms by 13.64% (Table 1) are consistent with a report from Wang et al. in which 300 µM ECH extended the mean lifespan of C. elegans by 15.82% (Wang et al. 2015).
Declines in body movement, pharyngeal pumping, and reproductive capacity during the aging process have been well described in C. elegans (Huang et al. 2004). Our results showed that ECH-mediated lifespan extension did not affect these age-related physiological functions (Fig. 2). In agreement with our results, other reports have previously indicated that ECH does not significantly alter the feeding behavior or reproductive capacity of C. elegans (Wang et al. 2015).
Lifespan and stress resistance are tightly interrelated (Larsen 1993; Kenyon 2010). Indeed, prolonged lifespan of C. eleganscorrelates with enhanced stress tolerance against different biological, chemical, and physical stressors (Morley and Morimoto 2004). In this sense, and in accordance with the lifespan-prolonging effect produced by ECH, we found a strong enhancement of the thermotolerance of C. elegans treated with ECH (Fig. 3a). Heat stress in C. elegans is accompanied by generation of oxidative stress (Finkel and Holbrook 2000), and previous studies have shown that the longevity-promoting activity of natural products is closely linked with their antioxidant activities (Abbas and Wink 2009; Kampkotter et al. 2008; Chen et al. 2013). Importantly, ECH was able to significantly alleviate the lethality of H2O2-induced oxidative stress (Fig. 3b). Increased ROS accumulation can cause damage to proteins, DNA, and lipids, resulting in acceleration of aging and age-related diseases (Finkel and Holbrook 2000). Importantly, in our study, ECH exhibited strong scavenging activity of intracellular ROS using an H2DCF-DA fluorescent probe (Fig. 4a). Notably, fat accumulation is also correlated with ROS levels (Singh et al. 2008). Supporting this point, we observed that ECH with strong ROS scavenging activity also significantly reduced fat accumulation in C. elegans using Nile red staining (Fig. 4b). Thus, these findings indicated that the lifespan extension produced by ECH might be attributed to the enhancement of stress resistance in worms. More intriguingly, we observed that ECH failed to extend the lifespan of mev-1 (kn-1) mutants (Fig. 5), which exhibit ROS overproduction and declined longevity (Ishii et al. 1998). This finding highly suggests that the increased resistance to oxidative stress produced by ECH to yield lifespan extension is partially dependent on endogenous signaling pathways other than direct antioxidant mechanisms.
Next, to further explore mechanisms underlying the longevity-prolonging effect of ECH, we focused on the DR pathway, an important longevity-regulating pathway in C. elegans, yeast, and Drosophila (Walker et al. 2005; Jiang et al. 2000; Kannan and Fridell 2013). The C. eleganseat-2 (ad1116) mutant is a long-lived worm that has been used as a genetic DR model (McKay et al. 2004). Consistently, we found that 200 µM ECH did not increase the lifespan of eat-2 (ad1116) mutants. Instead, mean lifespan was decreased by 10.61% (Fig. 6a); thus, the enhancement of lifespan produced by ECH relies on DR pathways, and 200 µM ECH is somehow toxic to eat-2 (ad1116) mutants. Because ECH did not decrease pharyngeal pumping rate or food intake (Fig. 2c, d; Fig. S3), we conclude that ECH increases longevity through the DR pathway without mimicking DR. Moreover, the current results demonstrate that 200 µM ECH extended the lifespan of sir-2.1 (ok434) mutants by 16.77% (Fig. 6b). This indicates that the longevity effect of ECH in worms was independent of sir-2.1, despite sir-2.1 encoding a deacetylase that extends lifespan during DR (Testa et al. 2014). Taken together, these results indicated that ECH exertinglongevity activity relies on DR pathways, but independent of sir-2.1.
The lifespan of C. elegans is subject to regulation by conserved signaling pathways and transcription factors that sense stress, environmental cues, and nutrient availability (Donato et al. 2017). Apart from the DR pathway, the insulin/IGF signaling (IIS) pathway is another conserved central controller for regulation of longevity in C. elegans (Kenyon 2010; Murphy et al. 2003). In this pathway, daf-2 encodes the receptor, an ortholog of mammalian insulin and IGF-1. In the presence of ligands, DAF-2 activates downstream genes and regulates DAF-16, a member of the FOXO family of transcription factors. Upon entering the nucleus, DAF-16 modulates target genes involved in lifespan regulation and stress resistance (Mukhopadhyay et al. 2006). Notably, the positive lifespan effect of ECH on wild-type C. elegans was abolished in both daf-2 (e1370) and daf-16 (mu86) mutants (Fig. 7a, b), suggesting the IIS pathway is required for lifespan extension produced by ECH. Furthermore, we observed that ECH significantly induced DAF-16 nuclear translocation in the TJ356 mutant worm carrying a daf-16::GFP transgene (Fig. 7c, d). Importantly, nuclear translocation of DAF-16 is a key step in the IIS pathway, as it regulates the transcription of a number of longevity- and stress tolerance-associated genes (Su and Wink 2015). Among the multiple targets of daf-16, sod-3 and hsp-16.2 are two major downstream effectors. Sod-3 plays an essential role in detoxification, while hsp-16.2 is implicated in resistance to proteotoxic stress by preventing aggregation of misfolded and unfolded proteins, thus contributing to the longevity of C. elegans (Murphy et al. 2003; Su and Wink 2015). Using quantitative real-time PCR, we found that ECH dramatically promoted the transcriptional levels of sod-3 and hsp-16.2 by approximately 72-fold and 89-fold, respectively (Fig. 7e, f). Furthermore, we found that ECH produced a modest increase in the protein levels of SOD-3 (1.22-fold) and HSP-16.2 (1.21-fold) using strains expressing SOD-3::GFP or HSP-16.2p::GFP (Fig. 7g, h). Together, these results demonstrated the impact of ECH on nematode lifespan and stress resistance through the IIS pathway.
HSF-1 has been reported to cooperate with DAF-16 to activate target genes, and can also promote longevity itself in C. elegans (Hsu et al. 2003; Morley and Morimoto 2004). However, our current results showed that ECH significantly extended the mean lifespan of not only wild-type worms, but also hsf-1 (sy441) mutant worms by 19.78% (Fig. 8), suggesting hsf-1 is not associated with the longevity-prolonging effect of ECH in C. elegans.
Finally, with modern increases in life expectancy, age-related neurodegenerative disorders represent a growing health concern. A considerable amount of research has shown that age-related diseases are closely related with aging and oxidative stress (Jiang et al. 2016), and focus on finding botanical compounds with longevity effects to prevent age-related diseases, especially neurodegenerative disorders (Chen et al. 2014; Pan et al. 2012). Here, we found that ECH remarkably increased the CI of C. elegans (Fig. 9), indicating that it can attenuate the age-dependent decline in physiological behavior. More importantly, ECH significantly increased the survival of CL4176 worms containing Aβ aggregates (Fig. 10), which are considered to be a pivotal pathological factor in AD (Luo 2006). Notably, the protective effect of ECH on Aβ-induced toxicity was approximately equal to that of ginkgolide A, a well-known agent with positive effects for AD (Wu et al. 2006). However, ECH treatment did not diminish PolyQ accumulation (Fig. 11), which is considered a pathological factor in Huntington’s disease (Morimoto 2008). Collectively, these results demonstrated a potential protective effect of ECH against Aβ-induced toxicity for nematodes.
In conclusion, our findings show that ECH is the major phenylethanoid glycoside with longevity activity in C. deserticola. We observed that ECH mediates lifespan extension via dietary restriction and IIS pathways to elicit significant improvement of stress resistance within worms and potential protective benefits against Aβ-induced toxicity. Thus, our results provide insight into ECH-mediated mechanisms of lifespan extension, which may yield potentially valuable therapeutic strategies for aging-related diseases such as Alzheimer’s disease.
References
Abbas S, Wink M (2009) Epigallocatechin gallate from green tea (Camellia sinensis) increases lifespan and stress resistance in Caenorhabditis elegans. Planta Med 75(3):216–221
Altintas O, Park S, Lee SJ (2016) The role of insulin/IGF-1 signaling in the longevity of model invertebrates, C. elegans and D. melanogaster. BMB Rep 49(2):81–92
Bamps S, Wirtz J, Savory FR, Lake D, Hope IA (2009) The Caenorhabditis elegans sirtuin gene, sir-2.1, is widely expressed and induced upon caloric restriction. Mech Ageing Dev 130(11–12):762–770
Bargmann CI, Hartwieg E, Horvitz HR (1993) Odoramt-selective genes and neurons mediate olfaction in C. elegans. Cell 74(3):515–527
Brenner S (1974) The genetics of Caenorhabditis elegans. Genetics 77(1):71–94
Cai RL, Yang MH, Shi Y, Chen J, Li YC, Qi Y (2010) Antifatigue activity of phenylethanoid-rich extract from Cistanche deserticola. Phytother Res 24:313–315
Chen M, Müller D, Richling E, Wink M (2013) Anthocyanin-rich purple wheat prolongs the life span of Caenorhabditis elegans probably by activating the DAF-16/FOXO transcription factor. J Agric Food Chem 61:3047–3053
Chen YJ, Brian O, Chen HZ, Xiao SY, Liu XJ, Monica D, Cao Y (2014) Huang, Q. R. Mechanism of longevity extension of Caenorhabditis elegans induced by pentagalloyl glucose isolated from Eucalyptus leaves. J Agric Food Chem 62:3422–3431
China Pharmacopoeia Committee (2010) Chinese pharmacopoeia, vol I. China Medical Science and Technology Press, Beijing, p 126
Donato V, Ayala FR, Cogliati S, Bauman C, Costa JG, Lenini C, Grau R (2017) Bacillus subtilis biofilm extends Caenorhabditis elegans longevity through downregulation of the insulin-like signalling pathway. Nat Commun 14332(8):1–15
Douglas PM, Baird NA, Simic MS, Uhlein S, McCormick MA, Wolff SC, Kennedy BK, Dillin A (2015) Heterotypic signals from neural HSF-1 separate thermotolerance from longevity. Cell Rep. 12:1196–1204
Fabian TJ, Johnson TE (1994) Production of age-synchronous mass cultures of Caenorhabditis elegans. J. Gerontol. 49(4):145–146
Finkel T, Holbrook NJ (2000) Oxidants, oxidative stress and the biology of ageing. Nature 408(6809):239–247
Fu GM, Pang HH, Wong YH (2008) Naturally occurring phenylethanoid glycosides: potential leads for new therapeutics. Curr Med Chem 15(25):2592–2613
Guarente L, Picard F (2005) Calorie restriction–the SIR2 connection. Cell 120(4):473–482
Guo YH, Cao LL, Zhao QS, Zhang LJ, Chen JJ, Liu BY, Zhao B (2016) Preliminary characterizations, antioxidant and hepatoprotective activity of polysaccharide from Cistanche deserticola. Int J Biol Macromol 93:678–685
Hesp K, Smant G, Kammenga JE (2015) Caenorhabditis elegans DAF-16/FOXO transcription factor and its mammalian homologs associate with age-related disease. Exp. Gerontl. 72:1–7
Honda Y, Tanaka M, Honda S (2010) Trehalose extends longevity in the nematode Caenorhabditis elegans. Aging Cell 9:558–569
Hsu AL, Murphy CT, Kenyon C (2003) Regulation of aging and age-related disease by DAF-16and heat-shock factor. Science 300(5622):1142–1145
Huang C, Xiong C, Kornfeld K (2004) Measurements of age-related changes of physiological processes that predict lifespan of Caenorhabditis elegans. Proc Natl Acad Sci USA 101(21):8084–8089
Ishii N, Fujii M, Hartman PS, Tsuda M, Yasuda K, Senoo-Matsuda N, Yanase S, Ayusawa D, Suzuki K (1998) A mutation in succinate dehydrogenase cytochrome b causes oxidative stress and ageing in nematodes. Nature 394(6694):694–697
Jiang Y, Tu PF (2009) Analysis of chemical constituents in Cistanche species. J Chromatogr A 1216:1970–1978
Jiang JC, Jaruga E, Repnevskaya MV, Jazwinski SM (2000) An intervention resembling caloric restriction prolongs life span and retards aging in yeast. FASEB J 14(14):2135–2137
Jiang TF, Sun Q, Chen SD (2016) Oxidative stress: a major pathogenesis and potential therapeutic targetof antioxidative agents in Parkinson’s disease and Alzheimer’s disease. Prog Neurobiol 147:1–19
Kaletta T, Hengartner MO (2006) Finding function in novel targets: C. elegans as a model organism. Nat Rev Drug Discov 5:387–399
Kampkotter A, Timpel C, Zurawski RF, Ruhl S, Chovolou Y, Proksch P, Watjen W (2008) Increase of stress resistance and lifespan of Caenorhabditis elegans by quercetin. Comp Biochem Phys B 149(2):314–323
Kannan K, Fridell YW (2013) Functional implications of Drosophila insulin-like peptides in metabolism, aging, and dietary restriction. Front Physiol 4:288
Kenyon CJ (2010) The genetics of ageing. Nature 464(7288):504–512
Lakowski B, Hekimi S (1998) The genetics of caloric restriction in Caenorhabditis elegans. Proc Natl Acad Sci USA 95(22):13091–13096
Larsen PL (1993) Aging and resistance to oxidative damage in Caenorhabditis elegans. Proc Natl Acad Sci USA 90(19):8905–8909
Li L, Tsao R, Yang R, Liou C, Young JC, Zhu H (2008) Isolation and purification of phenylethanoid glycosides from Cistanche deserticola by high-speed counter-current chromatography. Food Chem 108(2):702–710
Lin LW, Hsieh MT, Tsai FH, Wang WH, Wu CR (2002) Anti-nociceptive and anti-inflammatory activity caused by Cistanche deserticola in rodents. J Ethnopharmacol 83:177–182
Luo Y (2006) Alzheimer’s disease, the nematode Caenorhabditis elegans, and ginkgo biloba leaf extract. Life Sci 78(18):2066–2072
Matsuura T, Oikawa T, Wakabayashi T, Shingai R (2004) Effect of simultaneous presentation of multiple attractants on chemotactic response of the nematode Caenorhabditis elegans. Neurosci Res 48(4):419–429
McKay JP, Raizen DM, Gottschalk A, Schafer WR, Avery L (2004) eat-2 and eat-18 are required for nicotinic neurotransmission in the Caenorhabditis elegans pharynx. Genetics 166(1):161–169
Morimoto RI (2008) Proteotoxic stress and inducible chaperone networks in neurodegenerative disease and aging. Gene Dev 22(11):1427–1438
Morley JF, Morimoto RI (2004) Regulation of longevity in Caenorhabditis elegans by heat shock factor and molecular chaperones. Mol Biol Cell 15:657–664
Mukhopadhyay A, Oh SW, Tissenbaum HA (2006) Worming pathways to and from DAF-16/FOXO. Exp Gerontl 41(10):928–934
Murphy CT, McCarroll SA, Bargmann CI, Fraser A, Kamath RS, Ahring J, Li H, Kenyon C (2003) Genes that act downstream of DAF-16 to influence the lifespan of Caenorhabditis elegans. Nature 424(6946):277–283
Nan ZD, Zeng KW, Shi SP, Zhao MB, Jiang Y, Tu PF (2013) Phenylethanoid glycosides with anti-inflammatory activities from the stems of Cistanche deserticola cultured in Tarim desert. Fitoterapia 89:167–174
Nan ZD, Zhao MB, Zeng KW, Tian SH, Wang WN, Jiang Y, Tu PF (2016) Anti-inflammatory iridoids from the stems of Cistanche deserticola cultured in Tarim Desert. Chin J Nat Med 14(1):61–65
Pan MH, Lai CS, Tsai ML, Wu JC, Ho CT (2012) Molecular mechanisms for anti-aging by natural dietary compounds. Mol Nutr Food Res 56:88–115
Raynes R, Leckey BD, Nguyen K, Westerheide SD (2012) Heat shock and caloric restriction have a synergistic effect on the heat shock response in a sir2.1-dependent manner in Caenorhabditis elegans. J Biol Chem 287(34):29045–29053
Singh SP, Niemczyk M, Zimniak L, Zimniak P (2008) Fat accumulation in Caenorhabitis elegans triggered by the electrophilic lipid peroxidation product 4-hydroxynonenal (4-HNE). Aging 1:68–80
Su S, Wink M (2015) Natural lignans from Arctium lappa as antiaging agents in Caenorhabditis elegans. Phytochemistry 117:340–350
Testa G, Biasi F, Poli G, Chiarpotte E (2014) Calorie restriction and dietary restriction mimetics: a strategy for improving healthy aging and longevity. Curr Pharm Des 20(18):2950–2977
Walker G, Houthoofd K, Wanfleteren JR, Gems D (2005) Dietary restriction in C. elegans: from rate-of-living effects to nutrient sensing pathways. Mech Ageing Dev 126(9):929–937
Wang X, Zhang J, Lu L, Zhou L (2015) The longevity effect of echinacoside in Caenorhabditis elegans mediated through daf-16. Biosci Biotechnol Biochem 79(10):1676–1683
Wat E, Ng CF, Koon CM, Wong EC, Tomlinsin B, Lau CB (2016) The protective effect of Herba Cistanches on statin-induced myotoxicity in vitro. J Ethnopharmacol 190:68–73
Wu Y, Wu Z, Butko P, Christen Y, Lambert MP, Klein WL, Luo Y (2006) Amyloid-beta-induced pathological behaviors are suppressed by Ginkgo biloba extract EGb 761 and ginkgolides in transgenic Caenorhabditis elegans. J Neurosci 26(50):13102–13113
Zhang K, Ma X, He W, Li H, Han S, Jiang Y, Wu H, Han L, Ohno T, Uotsu N, Yamaguchi K, Ma Z, Tu P (2014) Extracts of Cistanche deserticola can antagonize immunosenescence and extend lifespan in senescence-accelerated mouse prone 8 (SAM-P8) mice. Evid Based Complement Alternat Med 2014:601383
Acknowledgements
The authors thank the CGC Center (Minneapolis, MN, USA) and Prof. J. Fei at Tongji University for providing the worm culture. Financial support from the National Natural Science Foundation (Grant no. 31670347, 81001369 and 31170327), Shanghai Science and Technology Commission of Shanghai Municipality (No. 15401901000) is gratefully acknowledged.
Author information
Authors and Affiliations
Corresponding author
Electronic supplementary material
Below is the link to the electronic supplementary material.
Rights and permissions
About this article
Cite this article
Chen, W., Lin, HR., Wei, CM. et al. Echinacoside, a phenylethanoid glycoside from Cistanche deserticola, extends lifespan of Caenorhabditis elegans and protects from Aβ-induced toxicity. Biogerontology 19, 47–65 (2018). https://doi.org/10.1007/s10522-017-9738-0
Received:
Accepted:
Published:
Issue Date:
DOI: https://doi.org/10.1007/s10522-017-9738-0