Abstract
Circulating tumor cell (CTC) isolation has made positive impacts on metastatic detection and therapy analysis for cancer patients. Microfluidic-elasto-filtration (MEF) device based on the critical elasto-capillary number (Ca * e ) has been proposed to utilize the optimal multi-parameter conditions, including cell diameter (dc), the diameter of cylindrical filter pores (dp), nonlinear cell elasticity and hydrodynamic drag force, for selectively capturing CTCs and depleting white blood cells (WBCs). In this paper, we propose a novel two-degree-of-freedom nonlinear mass–damper–spring (m–c–k) model to predict the dynamic behaviors of CTCs and WBCs in a generic MEF device. This model enables the optimization of the device design to achieve extremely high CTC capture efficiency and WBC depletion efficiency. In particular, the function of nonlinear cell stiffness specific to different cell types and MEF’s pore diameters is first determined by finite element method with neo-Hookean hyperelastic model, based on which the mechanical behaviors of CTCs and WBCs in MEF devices are systematically studied. Herein, the predicted normalized deformations of a CTC and WBC as a function of Cae are used to determine the optimized Cae of 0.043, consistent with the experimental results from the fabricated MEF devices using MCF-7 cells (0.04 ± 0.006). In addition, the normalized cell diameter versus Cae phase diagram is proposed for the first time as a useful tool for design optimization of MEF devices and other microfiltration devices.
Similar content being viewed by others
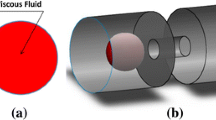
Avoid common mistakes on your manuscript.
1 Introduction
Cancer is still the worldwide leading cause of death, in which the metastasis leads more than 90% of deaths in cancer patients (Kaiser 2010; Wicha and Hayes 2011). Circulating tumor cells (CTCs) are the cancer cells that disseminate into the peripheral blood from primary or metastatic tumor sites. The detection of CTCs from blood can work as a liquid biopsy (Alix-Panabieres and Pantel 2013; Vaidyanathan et al. 2019), which is a relatively non-invasive way for cancer detection and therapy monitoring. Size-based microfiltration using membrane filters is a large category of CTC isolation methods that are based on physical property. Systems with various filter membranes made from track-etched polycarbonate (Desitter et al. 2011; Vona et al. 2000), Parylene (Harouaka et al. 2014; Zheng et al. 2007), nickel (Hosokawa et al. 2010), SOI (silicon on insulator) wafer (Lim et al. 2012), SU-8 photoresist (Adams et al. 2014) and PEGDA (Tang et al. 2014) have been developed as CTC isolation methods. However, the final purity of isolated CTCs was typically on the order of 10% or less in the size-based microfiltration devices using membrane filters (Harouaka et al. 2013), limiting the further molecular characterization of isolated CTCs (Chang et al. 2013).
To address these issues of microfiltration devices, we have proposed a novel microfluidic-elasto-filtration (MEF) method utilizing the elasto-capillary number (Cae) to separate CTCs from WBCs (Cong 2015, 2016). The Cae is defined as the ratio between the viscous force from fluid flow and cell elastic force at a specific fluid flow rate. With a systematic experimental study of different cancer cells which captured in the microfilters with different pore diameters and at different flow rates (Cong 2015, 2016), we identify, for the first time, the existence of the optimized Cae for MEF devices, Ca * e , which can achieve extremely high cell capture efficiency and the depletion of WBC/non-cancer cells. Since the Cae is a dimensionless number, the dynamic similitude theory (Munson et al. 2006) can enable the results of Ca * e (0.043) of cancer cell lines to be directly used for the isolation of CTCs from the human blood at the same Ca * e .
On the other hand, there is a lack of a simple and accurate model to directly guide the optimal design of Cae-based MEF and other microfiltration devices. One key challenge to construct a simplified model is how to describe the nonlinear cell mechanical behavior in MEF devices. To date, there are two main types of models for cell mechanics related to the MEF devices in the literature: micro/nanostructure-based models and continuum models (Chen 2014; Lim et al. 2006; Rodriguez et al. 2013). The micro/nanostructure-based cell models mainly focus on cell behaviors at the cytoskeleton-level, which are suitable for studying the local characteristics of cells. On the other hand, the continuum models, including cortical liquid drop models and solid models, focus on the global properties of cells. A two-dimensional plane strain model (solid model) was built to simulate the cell entry process into a constriction channel, considering Young’s modulus of the cell and the friction between the cell and channel wall (Luo et al. 2014). This work investigated more about the cell mechanical properties, which did not precisely predict the critical condition for a cell to enter or pass through the channel. In addition, there a few three-dimensional (3D) computational fluid dynamics (CFD) simulations (Aghaamoo et al. 2015; Zhang et al. 2014, 2015) developed to understand the cell behavior and pressure response when a cell passes through filter pores with different geometries and side-wall profiles. However, it is still challenging to directly convert these CFD simulation results into a general design rule for CTC isolation, due to the tremendous computational cost and the lack of experimental validations. There are other researchers using the Young–Laplace equation to describe the cell deformation under a certain pressure (McFaul et al. 2012; Tang et al. 2014) where the cell was considered as a liquid drop with a constant stiffness. Considering the large deformation occurs when a cell passes through a filter pore, such simplified equation is no longer suitable to describe the nonlinear behavior of cell stiffness.
In this paper, we propose a novel nonlinear two-degree-of-freedom (2-DOF) mass–damper–spring (m–c–k) system to describe the dynamic behavior of large cell deformation in a filter pore. Note that a linear 2-DOF model was used to study the adherent cell mass and growth by Park and his co-workers (Park et al. 2010). In general, the m–c–k model can reflect the cell viscoelastic property, in which the linear spring k and linear damping c are related to the elastic and viscous properties, respectively. Unfortunately, the simulation results of a linear m–c–k model failed to predict cell behaviors in MEF (Supplementary Fig. 1S). Therefore, we propose a nonlinear 2-DOF m–c–k model with a nonlinear cell stiffness as a function of cell deformation in a filter pore. The nonlinear stiffness function is determined by finite element method (FEM) with neo-Hookean hyperelastic model. The proposed nonlinear 2-DOF model can predict the capturing of CTCs and depletion of WBCs at different Cae and the diameter of cylindrical filter pores dp. An optimized Ca * e for CTC isolation is identified by the proposed model and verified by experimental results. Finally, the new nonlinear 2-DOF m–c–k model can predict the capture efficiency of CTCs which would provide a general guideline for the design of MEF devices and the other microfiltration systems for the isolation of CTCs and even other types of cancer cells.
2 Materials and methods
2.1 Finite element simulation for the nonlinear cell stiffness function
A two-dimensional (2D) plane stress model is used to describe a cell and a filter pore as shown in Fig. 1b, in which the cell is characterized as a neo-Hookean hyperelastic material which has been successfully used to study the nonlinear mechanical response of cells with large deformations (Caille et al. 2002; Dao et al. 2003; Ding et al. 2017; Zhou et al. 2005). The neo-Hookean hyperelastic model can predict the large deformation when the maximum strain is on the order of 50–60% (Caille et al. 2002; Peeters et al. 2005) and even 100% (Dao et al. 2003). The polycarbonate (PC) filters are assumed to be rigid surfaces and fixed, due to its high Young’s modulus of 2.0 GPa (Soloukhin et al. 2003) compared with that of a CTC (600 Pa) and a WBC (120 Pa) (Kuznetsova et al. 2007; Sajeesh et al. 2016). The side-wall of the filter pore is designed with the rounded corners with the radius of 2 µm for easy convergence, as the parametric study has shown that nonlinear cell stiffness is relatively insensitive to the radius of rounded corners (Supplementary Fig. 2S). A displacement is applied for pressing the cell to enter and pass through the filter pore at a uniform speed, which can be considered as a quasi-static process. As a result, the nonlinear cell stiffness in the x direction, k1(x) and k2(y) in the y direction can be determined as the ratio between the force and cell deformation at each time point.
Nonlinear two-degree-of-freedom (2-DOF) mass–damper–spring (m–c–k) model to predict the cell capturing in a microfluidic-elasto-filtration (MEF) device. a Dimensional analysis reveals the key dimensionless parameter for MEF devices, elasto-capillary number (Cae). b Finite element simulation based on the neo-Hookean hyperelastic model to determine the nonlinear cell stiffness as a function of cell deformation in x and y directions
In the FEM simulation, ANSYS Workbench 16.0 (ANSYS Inc, PA, USA) is used to study the mechanical responses of a CTC/WBC passing through filter pores with different diameters. According to the results of mesh convergence test (Supplementary Fig. 3S), a CTC and WBC are discretized into 3671 and 3215 quadrilateral elements, respectively. The neutrophil, constituting more than 60% of WBCs in human blood with relatively larger diameter compared with the other four WBC types (Bain 2006), is selected as a representative WBC in our simulation, while MCF-7 cell is selected as the CTC model due to their similar Young’s modulus measured by AFM (Atomic Force Microscope) indentation (Chen et al. 2013; Cross et al. 2007; Li et al. 2008) (Supplementary Table 1S).
2.2 Two-DOF nonlinear m–c–k model
The dimensionless parameter for characterizing the cell capturing in MEF is identified as Cae as shown in Fig. 1a. V is the average flow velocity defined as the filtration flow rate divided by the opening area (total area of all the filter pores) on the filter membrane and μ is the viscosity of fluid flow; σ0 is the initial cell stiffness. The cell behavior is described by a nonlinear 2-DOF m–c–k system to describe the cell deformation as the motion of its center of mass. In the x direction, perpendicular to the direction of fluid flow, a cell experiences a stretching shear force Fshear (White and Corfield 2006):
where rc (= 0.5dc; dc is the cell diameter) is the cell radius and x is the displacement of the m–c–k system. Considering the center of mass of the cell as the base point, the displacement of the m–c–k system, representing the deformation of a half cell in x direction, can be characterized by an ordinary differential equation (ODE):
In the y direction along the fluid flow, the cell experiences a hydrodynamic pressure loading Fpressure across the filter:
where rp (= 0.5dp) is the radius of a filter pore and ΔP is the pressure drop across a filter (Coumans et al. 2013):
where h is the height of the filter. Considering the contact surface between the cell and the filter pore as the base point, the deformation y of a cell can be characterized by the following ODE:
The cell mass m is estimated by the cell’s diameter and density (Grover et al. 2011), which was assumed to be constant during MEF (Supplementary Table 2S). The nonlinear cell stiffness of a CTC and WBC in x and y directions (i.e., k1,CTC(x), k2,CTC(y), k1,WBC(x) and k2,WBC(y)) have been determined by the FEM simulations for each pore diameter and fitted by six-order polynomial functions. The damping coefficient c was determined from the apparent cell viscosity µc of a CTC or WBC (Corbin et al. 2015; Xu and Shao 2008) (Supplementary Table 3S). The shear forces Fshear and pressure loading Fpressure can be expressed as a function of Cae by replacing μ and V using the definition of Cae. Hence, by solving the two ODEs of Eqs. (2) and (5) using the ODE solver of ode15s in MATLAB (2013b), the effects of Cae on the deformation of a cell pressed against a filter pore can be derived.
2.3 Chip fabrication
The detailed fabrication process of MEF chips can be found elsewhere (Cong 2015, 2016). In short, MEF chips were fabricated as a sandwich structure of two polydimethylsiloxane (PDMS) layers with a PC filter membrane (Whatman, UK) in-between (Supplementary Fig. 6S). The filter membrane was treated with (3-aminopropyl) triethoxysilane (APTES; Sigma-Aldrich, USA) to form a strong bonding with the PDMS layers. The effective filtration area was 1 cm2 with around 105 filter pores in each MEF chip.
2.4 Cell line and WBC sample preparation
Three types of mammalian cell lines, MCF-7 and HeLa cells (cancer cells) as well as HEK293 cells (non-cancer cells), were cultured using the high-glucose Dulbecco’s Modified Eagle Medium (DMEM) (Sigma-Aldrich, USA) supplemented with 10% fetal bovine serum (FBS) (Invitrogen, USA) and 1% penicillin–streptomycin (Invitrogen, USA), using sterile 10-cm2 flasks in an incubator at 5% (v/v) CO2 and at 37 °C. To harvest the cells, the sub-confluent monolayers were dissociated using 0.01% trypsin (Sigma-Aldrich, USA). Cell concentration was determined by manual counting using hemocytometer (Hausser Scientific, USA).
WBCs were collected from whole blood samples following a standard protocol (Estridge et al. 2000). Blood from healthy donors was collected using EDTA-coated tubes (Improve Medical Technology Co. Ltd, China). For each 3 mL of blood, 27 mL of RBC lysis buffer (Sigma-Aldrich, USA) was added and incubated for 20 min. The blood samples were then centrifuged for 5 min at 2000 rpm. After centrifugation, the supernatants were aspirated. The WBC pellets were re-suspended in 30 mL of RBC lysis buffer and centrifuged for 5 min at 2000 rpm to remove the residual RBCs. After removing the supernatant, the WBCs were harvested in phosphate-buffered saline (PBS). WBC concentration was also determined by manual counting using hemocytometer (Hausser Scientific, USA).
2.5 Experimental setup
Cell suspensions were injected into the MEF chips using a digital syringe pump at different flow rates. After filtration, the mammalian cells were labeled by 2 µg/mL of acridine orange/ethidium bromide (AO/EB) (Sigma-Aldrich, USA) in PBS, while the nuclei of WBC were labeled by DAPI (4′,6-diamidino-2-phenylindole) (Sigma-Aldrich, USA). The filtration experiments of either mammalian cells or WBCs were conducted under a series of Cae using MEF chips with different pore diameters, in which Cae was adjusted by either tuning the viscosity µ of fluid flow or the average flow velocity V (refer to the Supplementary Fig. 7S for more details). After filtration, the MEF chip was placed under a fluorescence microscope (BX41, Olympus, Japan) equipped with a CCD camera for cell imaging. The number of trapped cells in an MEF chip was counted by a digital image-processing program, CellProfiler (Carpenter et al. 2006). Capture efficiency (ηc) of mammalian cells was determined as the ratio between the number of captured cells on the filter and the total number of injected cells. The log-depletion of WBC (ηw-log) was defined as ηw-log = log(Ni/Nt), where Ni is the injected WBC number and Nt is the number of WBCs trapped on the filter.
3 Results and discussion
3.1 Nonlinear cell stiffness, k 1(x) and k 2(y)
The nonlinear cell stiffness when a CTC or WBC passing through a filter pore with different diameters were extracted from the FEM simulation, in which the maximum strain (~ 40%, Supplementary Fig. 8S) is within the range that the neo-Hookean hyperelastic model is tested to be reasonably accurate (Caille et al. 2002; Dao et al. 2003; Peeters et al. 2005). The cell stiffness in x direction k1(x), plotted as a function of cell deformation in Fig. 2a, keeps increasing when the cell deformation also increases. Such behavior is consistent with the strain hardening behavior of biological cells (Chen and Boyle 2014; Lee et al. 2007; Ye et al. 2014). Due to the higher Young’s modulus of CTCs, the magnitude of k1,CTC(x) is higher than that of k1,WBC(x). The k1(x) also turn out to be independent of the pore diameter since the cell deformation is relatively small (typically < 20% relative deformation).
The cell stiffness in y direction, k2(y), shown in Fig. 2b, increases first and then decreases after the cell enters the filter pore with a certain distance of ~ 3 to 4 µm. In an ideal case without friction between the cell and the surfaces of filter pore, the k2(y) reaches its maximum and starts to decrease when the displacement of the cell equals 5 µm, which is the half height of the filter membrane. This result is also consistent with other simulation results that when a cell passes through the half height of a filter pore, the net force on the cell will start to decrease and pull the cell out of the filter pore (Zhang et al. 2014), resulting in a reduction of cell stiffness k2(y). Since the friction factor between the cell and surfaces of the filter pore is considered as 0.01 in the FEM simulation (Milovanovic and Ma 2012), the reduction of k2(y) appears earlier than in the ideal case. The magnitude of k2,CTC(y) is also higher than k2,WBC(y) due to the higher Young’s modulus of CTCs. In conclusion, the behavior of k2(y) depends not only on cell types but also on the specific pore diameters.
3.2 Capture of CTCs at different Ca e(c)
The normalized CTC deformation y/h in y direction is plotted as a function of Cae(c) (Cae regarding to CTCs) in MEF chips with different pore diameters, shown as Fig. 3a. When Cae(c) increases, the y/h also increases meaning the CTC enters the filter pore under the increasing hydrodynamic pressure loading. When y/h is larger than 100%, the CTC is considered to pass through the pore. When y/h is smaller than 100%, the CTC is still trapped in the pore with an elongation in x direction due to the shear force, resulting in the enhancement for CTC capturing. Hence, the critical value of Cae(c) for enhanced CTC capturing can be identified as the value when y/h = 100%. As shown in Fig. 3a, the critical Cae(c) for enhancing the capture of CTC (Ca * e(c) ) was 0.197, 0.048, 0.024 and 0.012 for filter pores of 5, 8, 10 and 12 µm in diameter, respectively. Interestingly, after the CTC passed through the filter pore (y/h > 100%), the deformation of CTC becomes less sensitive to Cae(c) showing a much smaller slope of y/h, as there is no longer cell–pore interaction.
The capture of CTCs at different Cae(c) in MEF chips with different pore diameters. a Simulation results of the normalized cell deformation (y/h) as a function of Cae(c), in which the critical Cae(c) (Ca * e(c) ) for capturing CTCs on MEF devices with different pore diameters can be identified when y/h = 100%. b The identified Ca * e(c) can be verified by the experiment results in which the capture efficiency (ηc) of MCF-7 cells is either close to the peak value or keeps increasing when the experimental Cae(c) = Ca * e(c)
Experimentally, the ηc of MCF-7 cells (as the model of CTCs) was also tested as a function of Cae(c) using MEF chips with different pore diameters. As shown in Fig. 3b, the ηc first increased and then decreased with the increasing Cae(c) in MEF chips with 8, 10 and 12 µm pores. The ηc of the 5 µm pores was almost constant due to the upper limitation of cell deformation and thus not included for comparison (Cong 2015). The Ca * e(c) for each filter pore diameter in the simulation is either close to the Cae(c) at the peak value of ηc or within the Cae(c) range where ηc keeps increasing in the experiment. As the Ca * e(c) is predicted by the m–c–k model to enhance the capture of CTCs, the consistence with the experimental results is shown here.
3.3 Depletion of WBCs at different Ca e(w)
The depletion of WBCs is regarded as the opposite capturing of WBCs. To study the WBC capturing at different Cae(w) (Cae regarding to WBCs), the y/h of WBC is plotted as a function of Cae(w) on the filter with different pore diameters, shown as Fig. 4a. Using the same method for the identification of critical Ca * e(c) , the critical Cae(w) for enhanced capturing of WBCs, Ca * e(w) , can be identified as the value when y/h = 100%. As shown in Fig. 4a, Ca * e(w) was 0.08, 0.015, 0.01 and 0.007 for filter pores of 5, 8, 10 and 12 µm, respectively.
The depletion of WBCs at different Cae(w) in MEF chips with different pore diameters. a Simulation results of the y/h as a function of Cae(w), in which critical Ca * e(w) for capturing of WBCs on MEF devices with different pore diameters can be identified when y/h = 100%. b The identified Ca * e(w) can be verified by the experiment results in which the WBC depletion (ηw-log) is close to the minimum when the experimental Cae(w) = Ca * e(w)
To compare with the simulation results, the ηw-log of WBCs were also experimentally tested as a function of Cae(w) using MEF chips with different pore diameters. The ηw-log of the 5 µm pores were almost constant due to the upper limitation of cell deformation and thus not included for comparison (Cong 2015). As shown in Fig. 4b, the ηw-log of WBCs first decreased and then increased with the increasing Cae(w); the identified Ca * e(w) for each filter pore diameter located within the range that ηw-log close to the minimum. Thus, it is consistent between the simulation and experimental results that the ηw-log reaches the local minimum when Cae(w) is around Ca * e(w) , considering the Ca * e(w) is identified to enhance the capture of WBCs. As a result, when designing an MEF system for CTC isolation, it is advantageous to adjust the Cae(c) to be close to Ca * e(c) and the Cae(w) to be different from Ca * e(w) , which will enhance the ηc of CTCs and ηw-log of WBCs.
3.4 Predicting the capturing of other cell types
To generalize the simulation results, in addition to CTCs and WBCs, more simulations have been conducted to study the Ca * e(c) for the capturing of other cell types. Figure 5a shows the y/h of HEK293, MCF-7 and HeLa cells as a function of Cae(c) in MEF chips with 8 µm pores. Following the same method used in Figs. 3a and 4a, the Ca * e(c) for enhancing the capture of HEK293, MCF-7 and HeLa cells were identified as 0.037, 0.048 and 0.055, respectively. The Ca * e(c) for different cell types consistently located at the value of the peak ηc in experiment results is shown in Fig. 5b. Thus, this shows that the proposed nonlinear m–c–k model can be applied to predict the capturing of different cell types.
The capturing of three types of cells in MEF chips with 8 µm pores at different Cae(c). a Simulation results about the y/h of different types of cells as a function of Cae(c), in which the Ca * e(c) for enhancing the cell capturing can be identified when y/h = 100%. b The identified Ca * e(c) can be verified by the experiment results in which the ηc reaches the peak value when the experimental Cae(c) = Ca * e(c) for the three types of cells
3.5 Optimized Ca e for CTC isolation
In practical applications of MEF devices, CTCs and WBCs are suspended in the same sample solution in which they experience the same flow conditions during MEF. To reveal the Cae effect on isolating CTCs, the y/h of a CTC and WBC in a filter pore of 8 µm are plotted as a function of Cae(c) and Cae(w), respectively. Note that under the same flow conditions (µ and V), the Cae(w) is fivefold higher than the corresponding Cae(c) due to the initial stiffness of WBCs is fivefold lower than that of CTCs (Hochmuth et al. 1993; Kim et al. 2013). As shown in Fig. 6a, when Cae(c) and Cae(w) increase, the y/h of a WBC reaches 100% much faster than that of a CTC, indicating that the WBC can pass through the filter pore while the CTC will get trapped under proper flow conditions. An optimized Cae (Ca * e , with respect to the CTC, Cae(c)) of 0.043 can be determined at the inflection point of CTC-y/h curve before it reaches 100%, considering that the CTC-y/h becomes extremely sensitive to Cae(c) (with a large slope) when it becomes close to 100%, to avoid the loss of CTCs due to the error in tuning the Cae(c) during experiments. At Ca * e , the y/h for CTC is only ~ 50% indicating the CTC is trapped, while that of WBC has exceeded 100% already, meaning the WBC has passed through the filter pore. Hence, the CTC can be selectively captured by controlling the flow conditions in MEF to achieve the Cae(c) = Ca * e .
The identification and verification of the optimized elasto-capillary number Ca * e for the optimal CTC isolation performances through the proposed model, experiments and references. a The identified Ca * e of 0.043 using the proposed model to achieve high CTC capture efficiency and WBC depletion efficiency; b experimentally identified Ca * e of 0.04 ± 0.006 to achieve maximum capture efficiency of MCF7 cells and high depletion of WBCs; c verification of the identified Ca * e by comparing the operational Cae and performances among MEF and the other microfiltration systems (nickel-based filter, square; MEF chip, circle; SOI-wafer filter, triangle; Rarecells®, diamond)
The ηc of MCF-7 cells (as the model of CTCs) and ηw-log of WBCs have also been determined under a series of Cae(c) and Cae(w) in MEF chips with 8 µm pores. As shown in Fig. 6b, the ηc reaches a peak value when Cae(c) is 0.04 with an error of ± 0.006 based on the experimental error analysis, while the ηw-log continuous increases within the tested Cae(w) range. Since the isolation of CTCs from a typical blood sample deals with the mixture of CTCs and WBCs in a ratio as low as 1:107, it requires the MEF to gain the maximum ηc and simultaneously to achieve the ηw-log as high as possible. Based on this requirement, such Cae(c) ≅ 0.04 can be experimentally identified as the optimized Cae(c) that achieved the maximum capture efficiency of CTCs and optimized depletion efficiency of WBCs. This Cae(c) ≅ 0.04 is also very close to the identified Ca * e (= 0.043) from our model. This confirms that the proposed nonlinear m–c–k model is a good tool to guide the design of MEF chips with optimal performances in CTC isolation.
To further validate the existence of the Ca * e , the comparison of the operational Cae(c) and the corresponding performances among several microfiltration systems has been conducted. These systems are nickel-based filter (Hosokawa et al. 2010), Rarecells® ISET technique (Vona et al. 2000) and SOI-wafer filter (Lee et al. 2013) (summarized in Supplementary Table 4S). As shown in Fig. 6c, for different systems tested by the same cancer cell line, MCF-7 cells, and filters with 8 µm pores, the highest ηc and ηw-log are consistently achieved when the operational Cae(c) close to the Ca * e , while the ηc and ηw-log become lower when the operational Cae(c) deviates from the Ca * e . This comparison indicates that the Ca * e identified by the proposed 2-DOF nonlinear m–c–k model is also a generalized tool towards a microfiltration system with optimal performances for CTC isolation.
3.6 The generalized phase diagram of CTC capture efficiency
Based on the excellent agreement between the predicted ηc from our model and the experimental results, we apply our model to predict the capturing of a group of CTCs with different cell diameters following the size distribution of MCF-7 cells. To further generalize the results, another dimensionless parameter, the normalized cell diameter with respect to the pore diameter, d*= dc/dp, has been introduced. Therefore, we can calculate the ηc as a function of Cae(c) and d* (refer to section 11 of Supplementary materials) and construct the phase diagram as a contour plot which will be useful for the design optimization as shown in Fig. 7a.
The phase diagrams as the contour plot of the capture efficiency ηc as a function of Cae(c) and the normalized cell diameter d*. a The phase diagram generated by the simulation results indicates that the optimized Ca * e of 0.043 can achieve the maximum ηc; b the phase diagram derived from the experiment data shows that, for each d*, ηc of MCF-7 cells reaches the maximum when Cae(c) = 0.04 which is regarded as the optimized Ca * e in MEF experiments
Figure 7b is the experimental ηc-phase diagram of MCF-7 cells (as the model of CTCs) which shows good agreement with the theoretical counterpart in Fig. 7a. For each d*, there are three dominant Cae(c) effects on ηc. When the Cae(c) is from 0 to 0.02, the ηc decreases when the Cae(c) increases as a result of the increasing hydrodynamic pressure loading in y direction. In the moderate range of Cae(c), from 0.02 to 0.04, the ηc increases as the Cae(c) increases. This is due to the deformation in x direction becomes more significant to enhance the trapping of cells (with the elongation from 2 to 5 µm in diameter, see Supplementary Fig. 10S) when y/h is smaller than 100%, though the hydrodynamic pressure loading continues to push the cell to pass through the pore. When the Cae(c) is larger than 0.04, the ηc decreases again due to the increasing hydrodynamic pressure loading. Though the deformation in x direction keeps increasing, the cells will escape from the pores due to the heavy pressure loading (y/h > 100%). Thus, the optimized Ca * e (with respect to CTC Cae(c)) of 0.043 and 0.04 can also be identified for the maximum ηc from the phase diagrams of simulation and experimental results, respectively. This agreement shows that the proposed nonlinear 2-DOF m–c–k model is very useful to quantitatively predict the capture efficiency of a group of CTCs.
4 Conclusions
We propose a novel nonlinear 2-DOF m–c–k model to predict the cell capturing in MEF devices considering the large deformation of cells. The predicted capture efficiency of CTC and depletion efficiency of WBC at different Cae and dp reveal the existence of the optimized Cae (Ca * e ) of 0.043 to achieve the optimized CTC isolation in MEF devices, consistent with the experimental Ca * e of 0.04 with respect to CTCs (MCF-7 cells as the model in the experiments) using the fabricated MEF devices. The proposed model has been used to predict the Ca * e(c) for the other two types of cells, HEK293 and HeLa cells, respectively, resulting in a good agreement with the experimental results. We also propose the generalized phase diagram of CTC capture efficiency which can be a useful tool for design optimization.
Since the Cae is a dimensionless number, the dynamic similitude theory in fluid mechanics can enable the results of Ca * e to be used for the optimization of the other microfiltration devices. In addition, this Ca * e can also be used for clinical experiments, i.e., the isolation of CTCs in human blood samples at the same Ca * e (such as 0.04 for breast cancer) to achieve excellent CTC capture efficiency and WBC depletion efficiency, which will be reported in a new paper in the near future.
References
Adams DL et al (2014) The systematic study of circulating tumor cell isolation using lithographic microfilters. RSC Adv 9:4334–4342. https://doi.org/10.1039/C3RA46839A
Aghaamoo M, Zhang Z, Chen X, Xu J (2015) Deformability-based circulating tumor cell separation with conical-shaped microfilters: concept, optimization, and design criteria. Biomicrofluidics 9:034106. https://doi.org/10.1063/1.4922081
Alix-Panabieres C, Pantel K (2013) Circulating tumor cells: liquid biopsy of cancer. Clin Chem 59:110–118. https://doi.org/10.1373/clinchem.2012.194258
Bain BJ (2006) Blood cells: a practical guide, 4th edn. Blackwell Publishing Asia Pty Ltd., Australia
Caille N, Thoumine O, Tardy Y, Meister J-J (2002) Contribution of the nucleus to the mechanical properties of endothelial cells. J Biomech 35:177–187. https://doi.org/10.1016/S0021-9290(01)00201-9
Carpenter A et al (2006) Cell profiler: image analysis software for identifying and quantifying cell phenotypes. Genome Biol 7:R100
Chang H, Zhou P, Xie Z, Gong X, Yang Y, Yuan W (2013) Theoretical modeling for a six-DOF vortex inertial sensor and experimental verification. J Microelectromech Syst 22:1100–1108
Chen J (2014) Nanobiomechanics of living cells: a review. Interface Focus 4:20130055
Chen M, Boyle FJ (2014) Investigation of membrane mechanics using spring networks: application to red-blood-cell modelling. Mater Sci Eng C 43:506–516. https://doi.org/10.1016/j.msec.2014.07.043
Chen C-L et al (2013) Single-cell analysis of circulating tumor cells identifies cumulative expression patterns of EMT-related genes in metastatic prostate cancer. Prostate 73:813–826. https://doi.org/10.1002/pros.22625
Cong Z et al (2015) The capillary number effect on the capture efficiency of cancer cells on composite microfluidic filtration chips. In: Micro electro mechanical systems (MEMS), 2015 28th IEEE international conference on, 18–22 January 2015, pp 459–462. https://doi.org/10.1109/memsys.2015.7050989
Cong Z et al (2016) Capillary number effect on the depletion of leucocytes of blood in microfiltration chips for the isolation of circulating tumor cells. In: Proceedings of the 11th annual IEEE international conference on nano/micro engineered and molecular systems (IEEE-NEMS 2016), Matsushima Bay and Sendai MEMS City, Japan, p 1
Corbin EA, Kong F, Lim CT, King WP, Bashir R (2015) Biophysical properties of human breast cancer cells measured using silicon MEMS resonators and atomic force microscopy. Lab Chip 15:839–847. https://doi.org/10.1039/C4LC01179A
Coumans FAW, van Dalum G, Beck M, Terstappen LWMM (2013) Filtration parameters influencing circulating tumor cell enrichment from whole blood. PLoS One 8:e61774. https://doi.org/10.1371/journal.pone.0061774
Cross SE, Jin Y-S, Rao J, Gimzewski JK (2007) Nanomechanical analysis of cells from cancer patients. Nat Nano 2:780–783
Dao M, Lim CT, Suresh S (2003) Mechanics of the human red blood cell deformed by optical tweezers. J Mech Phys Solids 51:2259–2280. https://doi.org/10.1016/j.jmps.2003.09.019
Desitter I et al (2011) A new device for rapid isolation by size and characterization of rare circulating tumor cells. Anticancer Res 31:427–441
Ding Y, Xu G-K, Wang G-F (2017) On the determination of elastic moduli of cells by AFM based indentation. Sci Rep 7:45575. https://doi.org/10.1038/srep45575
Estridge BH, Reynolds AP, Walters NJ (2000) Basic medical laboratory techniques. Delmar Cengage Learning, Albany, NY
Grover WH, Bryan AK, Diez-Silva M, Suresh S, Higgins JM, Manalis SR (2011) Measuring single-cell density. PNAS 108:10992–10996. https://doi.org/10.1073/pnas.1104651108
Harouaka RA, Nisic M, Zheng S-Y (2013) Circulating tumor cell enrichment based on physical properties. JALA 18:455–468. https://doi.org/10.1177/2211068213494391
Harouaka RA et al (2014) Flexible micro spring array device for high-throughput enrichment of viable circulating tumor cells. Clin Chem 60:323–333. https://doi.org/10.1373/clinchem.2013.206805
Hochmuth RM, Ting-Beall HP, Beaty BB, Needham D, Tran-Son-Tay R (1993) Viscosity of passive human neutrophils undergoing small deformations. Biophys J 64:1596–1601
Hosokawa M, Hayata T, Fukuda Y, Arakaki A, Yoshino T, Tanaka T, Matsunaga T (2010) Size-selective microcavity array for rapid and efficient detection of circulating tumor cells. Anal Chem 82:6629–6635. https://doi.org/10.1021/ac101222x
Kaiser J (2010) Cancer’s circulation problem. Science 327:1072–1074. https://doi.org/10.1126/science.327.5969.1072
Kim J-D, Waleed M, Lee Y-G (2013) Stiffness measurement of a biomaterial by optical manipulation of microparticle. In: Proceedings of SPIE 8595: 85951G. https://doi.org/10.1117/12.2005544
Kuznetsova TG, Starodubtseva MN, Yegorenkov NI, Chizhik SA, Zhdanov RI (2007) Atomic force microscopy probing of cell elasticity. Micron 38:824–833. https://doi.org/10.1016/j.micron.2007.06.011
Lee SS, Antaki JF, Kameneva MV, Dobbe JG, Hardeman MR, Ahn KH, Lee SJ (2007) Strain hardening of red blood cells by accumulated cyclic supraphysiological stress. Artif Organs 31:80–86. https://doi.org/10.1111/j.1525-1594.2007.00344.x
Lee HJ et al (2013) Efficient isolation and accurate in situ analysis of circulating tumor cells using detachable beads and a high-pore-density filter. Angew Chem Int Ed 52:8337–8340. https://doi.org/10.1002/anie.201302278
Li QS, Lee GYH, Ong CN, Lim CT (2008) AFM indentation study of breast cancer cells. BBRC 374:609–613. https://doi.org/10.1016/j.bbrc.2008.07.078
Lim C, Zhou E, Quek S (2006) Mechanical models for living cells—a review. J Biomech 39:195–216
Lim LS et al (2012) Microsieve lab-chip device for rapid enumeration and fluorescence in situ hybridization of circulating tumor cells. Lab Chip 12:4388–4396. https://doi.org/10.1039/C2LC20750H
Luo Y et al (2014) A constriction channel based microfluidic system enabling continuous characterization of cellular instantaneous Young’s modulus. Sens Actuators B Chem 202:1183–1189
McFaul SM, Lin BK, Ma H (2012) Cell separation based on size and deformability using microfluidic funnel ratchets. Lab Chip 12:2369–2376. https://doi.org/10.1039/C2LC21045B
Milovanovic L, Ma H (2012) Method for measurement of friction forces on single cells in microfluidic devices. Anal Methods 4:4303–4309. https://doi.org/10.1039/C2AY25740H
Munson BR, Young DF, Okiishi TH, Huebsch WW (2006) Fundamentals of fluid mechanics, vol 69. Wiley, Hoboken, p 520
Park K et al (2010) Measurement of adherent cell mass and growth. PNAS 107:20691–20696. https://doi.org/10.1073/pnas.1011365107
Peeters EAG, Oomens CWJ, Bouten CVC, Bader DL, Baaijens FPT (2005) Mechanical and failure properties of single attached cells under compression. J Biomech 38:1685–1693. https://doi.org/10.1016/j.jbiomech.2004.07.018
Rodriguez ML, McGarry PJ, Sniadecki NJ (2013) Review on cell mechanics: experimental and modeling approaches. Appl Mech Rev 65:060801
Sajeesh P, Raj A, Doble M, Sen AK (2016) Characterization and sorting of cells based on stiffness contrast in a microfluidic channel. RSC Adv 6:74704–74714. https://doi.org/10.1039/C6RA09099K
Soloukhin VA, Brokken-Zijp JCM, van Asselen OLJ, de With G (2003) Physical aging of polycarbonate: elastic modulus, hardness, creep, endothermic peak, molecular weight distribution, and infrared data. Macromolecules 36:7585–7597. https://doi.org/10.1021/ma0342980
Tang Y, Shi J, Li S, Wang L, Cayre YE, Chen Y (2014) Microfluidic device with integrated microfilter of conical-shaped holes for high efficiency and high purity capture of circulating tumor cells. Sci Rep 4:6052. https://doi.org/10.1038/srep06052
Vaidyanathan R, Soon RH, Zhang P, Jiang K, Lim CT (2019) Cancer diagnosis: from tumor to liquid biopsy and beyond. Lab Chip 19:11–34. https://doi.org/10.1039/C8LC00684A
Vona G et al (2000) Isolation by size of epithelial tumor cells: a new method for the immunomorphological and molecular characterization of circulating tumor cells. Am J Pathol 156:57–63
White FM, Corfield I (2006) Viscous fluid flow, vol 3. McGraw-Hill, New York
Wicha M, Hayes D (2011) Circulating tumor cells: not all detected cells are bad and not all bad cells are detected. J Clin Oncol 29:1508–1511
Xu G, Shao J-Y (2008) Human neutrophil surface protrusion under a point load: location independence and viscoelasticity. Am J Physiol Cell Physiol 295:C1434–C1444. https://doi.org/10.1152/ajpcell.00136.2008
Ye S, Ng Y, Tan J, Leo H, Kim S (2014) Two-dimensional strain-hardening membrane model for large deformation behavior of multiple red blood cells in high shear conditions. Theor Biol Med Model 11:19
Zhang Z, Xu J, Hong B, Chen X (2014) The effects of 3D channel geometry on CTC passing pressure—towards deformability-based cancer cell separation. Lab Chip. https://doi.org/10.1039/c4lc00301b
Zhang Z, Chen X, Xu J (2015) Entry effects of droplet in a micro confinement: implications for deformation-based circulating tumor cell microfiltration. Biomicrofluidics 9:024108. https://doi.org/10.1063/1.4916645
Zheng S, Lin H, Liu JQ, Balic M, Datar R, Cote RJ, Tai YC (2007) Membrane microfilter device for selective capture, electrolysis and genomic analysis of human circulating tumor cells. J Chromatogr A 1162:154–161
Zhou EH, Lim CT, Quek ST (2005) Finite element simulation of the micropipette aspiration of a living cell undergoing large viscoelastic deformation. Mech Adv Mater Struct 12:501–512. https://doi.org/10.1080/15376490500259335
Acknowledgements
The authors would like to acknowledge the support of a Grant from HKUST-BME Division, the Grants from NSFC (nos. 81372274, 8141101080 and 11702236), Guangzhou Science and Technology Innovation Commission (no. 201704030101), Guangdong Science and Technology Grant (no. 2017B050506001) and Hong Kong ITF Grant (no. GHP/076/17GD).
Author information
Authors and Affiliations
Corresponding author
Additional information
Publisher's Note
Springer Nature remains neutral with regard to jurisdictional claims in published maps and institutional affiliations.
Electronic supplementary material
Below is the link to the electronic supplementary material.
Rights and permissions
About this article
Cite this article
Luo, H., Zhao, C., Song, K. et al. A nonlinear two-degree-of-freedom mass–damper–spring model to predict the isolation of circulating tumor cells in microfluidic-elasto-filtration devices. Microfluid Nanofluid 23, 72 (2019). https://doi.org/10.1007/s10404-019-2240-z
Received:
Accepted:
Published:
DOI: https://doi.org/10.1007/s10404-019-2240-z