Abstract
Ozone (O3) pollution and the availability of nitrogen (N) and phosphorus (P) in the soil both affect plant photosynthesis and chlorophyll (Chl) content, but the interaction of O3 and nutrition is unclear. We postulated that the nutritional condition changes plant photosynthetic responses to O3. An O3-sensitive poplar clone (Oxford) was subject to two N levels (N0, 0 kg N ha− 1; N80, 80 kg N ha− 1), two P levels (P0, 0 kg P ha− 1; P80, 80 kg P ha− 1) and three levels of O3 exposure (ambient concentration, AA; 1.5 × AA; 2.0 × AA) over a growing season in an O3 free air controlled exposure (FACE) facility. The daily change of leaf gas exchange and dark respiration (Rd) were investigated at mid-summer (August). Chl a fluorescence was measured three times in July, August and September. At the end of the growing season, Chl content was measured. It was found that Chl content, the maximum quantum yield (Fv/Fm), Chl a fluorescence performance index (PI) and gas exchange were negatively affected by elevated O3. Phosphorus may mitigate the O3-induced reduction of the ratio of photosynthesis to stomatal conductance, while it exacerbated the O3-induced loss of Fv/Fm. Nitrogen alleviated negative effects of O3 on Fv/Fm and PI in July. Ozone-induced loss of net photosynthetic rate was mitigated by N in medium O3 exposure (1.5 × AA). However, such a mitigation effect was not observed in the higher O3 level (2.0 × AA). Nitrogen addition exacerbated O3-induced increase of Rd suggesting an increased respiratory carbon loss in the presence of O3 and N. This may result in a further reduction of the net carbon gain for poplars exposed to O3.
Similar content being viewed by others
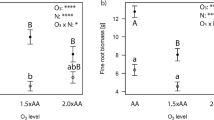
Explore related subjects
Discover the latest articles, news and stories from top researchers in related subjects.Avoid common mistakes on your manuscript.
Introduction
Tropospheric ozone (O3) pollution is a serious concern to plant health in many areas of the world (Matyssek et al. 2013; Mills et al. 2018). Ozone pollution has been rising continuously since the preindustrial age (Vingarzan 2004), although control measures on precursor emission are now successful in some areas of the world, such as North America (Cooper et al. 2014) and Europe (Paoletti et al. 2014; Sicard et al. 2013).
Nitrogen oxides are primary sources for O3 formation in the atmosphere (Crutzen 1970). In the United States, Ollinger et al. (2002) reported that high O3 concentration was often observed in regions where nitrogen (N) deposition from the atmosphere was high. Nitrogen input generally stimulates plant growth (Wooliver et al. 2017). Another important macronutrient influencing the change of plant growth is phosphorus (P) (Marchner 2011). Phosphorus plays a role in photosynthesis, energy storage and transfer, cell division and the development of new tissue (Marchner, 2011). Phosphorus contents in soils are usually determined by land use and in particular fertilization practices, while global P deposition from the atmosphere represents a relatively small amount (3–3.5 Mton year− 1) mostly derived from wind-eroded particles and biomass burning aerosols (Peñuelas et al. 2012).
Poplars are widely used for wood production and as a model plant in plant physiology (Christersson 2010), and are generally susceptible to oxidative stress due to O3 exposure (Hoshika et al. 2018). Poplar plantation is established in various soil conditions with low- (e.g., volcanic ash soils, Hoosbeek et al. 2004) and high-nutritional availability (e.g., agricultural field soils, Arevalo et al. 2011). Nutrient-rich conditions may reduce the production of secondary metabolites in relation to plant resistance to abiotic and/or biotic stress, i.e., the carbon-nutrient balance hypothesis (Bryant et al. 1983) thus changing the metabolic capacity for coping with O3 stress (Schulze 1989), although this hypothesis explains mainly behavior of phenolic compounds (Koricheva 2002). Braun et al. (2017) reported that the basal area increment in beech and Norway spruce may be limited by O3 and that a significant interaction exists with nutrition such as N and P. However, our knowledge of plant responses to O3 under various nutritional conditions is still limited. Only a few studies have investigated the effects of different N loads on O3 sensitivity in terms of photosynthetic traits, with mitigating (Fusaro et al. 2017; Häikiö et al. 2007; Watanabe et al. 2006), detrimental (Maurer et al. 1997; Yamaguchi et al. 2007) and neutral effects (Feng et al. 2011; Harmens et al. 2017). To our knowledge, the interaction between N, P and O3 on photosynthetic traits has never been tested.
The aim of the present work was to explore the interactive impacts of O3, N and P on the photosynthetic traits (gas exchange, chlorophyll (Chl) a fluorescence and content) of an O3-sensitive poplar clone (Populus maximoviczii Henry × Populus berolinensis Dippel) upon exposure to ambient, 1.5 fold ambient and twice ambient O3 conditions in open air. In our previous paper with the same poplar clone (Zhang et al. 2018), we showed that O3-induced biomass decline was greater at high N supply, while P alleviated such biomass losses, but not under high N supply. We postulated that higher nutritional availability may exacerbate the negative impacts of O3 on photosynthetic traits.
Materials and methods
Plant material, ozone exposure and nutritional treatments
Rooted cuttings of the Oxford poplar clone (Populus maximoviczii Henry × Populus berolinensis Dippel) were propagated in December 2015, and transplanted into 10 L plastic pots filled with a mixture of commercial river sand, sphagnum peat moss and soil, in a proportion 1: 1: 1 (v: v: v) in April 2016. Soil was collected in a semi-natural area nearby the experimental site (43° 46′ 56″N, 11° 10′ 24″E), characterized by a sandy-loam texture slightly acidic. The plants were irrigated to field capacity every 2–3 days to avoid water stress.
Plants were fumigated from 1st May to 1st October, 2016 (24 h per day) under three O3 levels: ambient air concentration (AA), 1.5 × AA and 2.0 × AA, in a free air controlled exposure (FACE) system located in Florence, Italy (43° 48′ 59″N, 11° 12′ 01″E, 55 m a.s.l.). More technical information about this facility are available in Paoletti et al. (2017). There were three replicated blocks in each O3 concentration, and 12 plants in each block. Three of the plants in each block were randomly subject to each of four nutritional treatments as explained below. Ozone was produced by a generator (TGOC13X, Triogen ltd., Glasgow, UK), mixed with ambient air and distributed into the O3 FACE using a system of 25 Teflon tubes in each block hanging from a fixed grid above the plants (Paoletti et al. 2017). Ozone concentration at plant height was continuously measured by O3 analyzers (Model 202, 2B Technologies Inc., Boulder, Colorado, USA) and adjusted to the target level by a PID system. The AOT40 (Accumulated Ozone exposure over a Threshold of 40 ppb) during the experimental period was 14.4 ppm.h in AA, 43.8 ppm.h in 1.5 × AA, and 71.1 ppm.h in 2.0 × AA (Zhang et al. 2018).
Two N concentrations (N0: 0 kg N ha− 1, i.e. 0 mg N seedling− 1; N80: 80 kg N ha− 1, i.e. 392.5 mg N seedling− 1) were added to the pot soil using NH4NO3 (0 and 5 mM solution) according to Thomas et al. (1994). The level of N80 may be considered as a realistic N deposition because the background deposition in some areas, such as the Sichuan basin in China and the California central valley in USA, may be higher than 80 kg N ha− 1 yr− 1 (Fenn et al. 2003; Peng et al. 2017). Two P concentrations (P0: 0 kg P ha− 1, i.e. 0 mg P seedling− 1; P80: 80 kg P ha− 1, i.e. 392.5 mg P seedling− 1) were supplied to the pot soil as KH2PO4 (0 and 1.0 mM solution) according to Lewis and Strain (1996). Based on P affinity constant and adsorption maxima, these levels of P were selected to simulate a realistic increase in soil available P. In total, there were four combinations of nutrient treatment, i.e. N0P0, N0P80, N80P0, N80P80. In detail, NH4NO3 or KH2PO4 solution (200 ml) with different concentrations as described above were added into the soil twice a week during the whole treatment period, with an aim of reaching the target concentrations at the end of the experiment, for a total of 11 applications. In order to maintain the same level of K among all treatments, KCl was added into the soil that did not receive KH2PO4 (Tissue and Lewis 2010).
The soil N and P concentrations and the N:P ratio of leaves are shown in our previous paper (Zhang et al. 2018). Soil N concentration (mean ± SE) in N0 was 1.7 ± 0.1 g kg− 1, and that in N80 treatments was 2.7 ± 0.1 g kg− 1. These are in agreement with normal soil N ranges (0.2 to 5 g kg− 1, Bowen 1979). Soil P concentrations (mean ± SE) were 0.5 ± 0.1 g kg− 1 in P0 and 1.0 ± 0.1 g kg− 1 in P80, that are within the range of native P in soils (usually 0.5 to 0.8 g kg− 1, and the maximum is found as 1.0 to 1.3 g kg− 1, Stevenson and Cole 1999). Leaf N:P ratios (mean ± SE) were 12.1 ± 1.1 at N0P0, 8.3 ± 1.1 at N0P80, 18.8 ± 1.5 at N80P0, and 10.6 ± 1.4 at N80P80 (Zhang et al. 2018). Koerselman and Meuleman (1996) suggested an optimal aboveground biomass N:P ratio of 14–16.
Gas exchange and Chl a fluorescence measurements
Daily course of leaf gas exchange was measured for fully-expanded sun leaves (5-8th order from the tip of terminal shoots, flushed in July 2016), using a portable infra-red gas analyzer (CIRAS2, PP-systems, UK). No typical symptoms of senescence (e.g., yellowing) were observed, although some O3-like visible foliar injury (dark stippling in the interveinal area) was found in 1.5 × AA and 2.0 × AA. Measurements were carried out in 1 to 3 plants per plot every 3 h (morning: 9:00, noon: 12:00, afternoon: 15:00) on 25 August 2016. Leaves in the cuvette were oriented so as to be fully and directly exposed to sunlight. Net photosynthetic rate (PN) and stomatal conductance (gs) were measured at a CO2 concentration (mean ± SE) of 385.9 ± 0.5 µmol mol− 1. The temperature in the cuvette was adjusted manually to the ambient conditions, which were measured by a thermometer under shaded condition at plant canopy height. Relative humidity in the cuvette was adjusted to be similar to the ambient humidity. Dark respiration rate was determined in the laboratory by putting the plants in shade for 30 min. The CO2 efflux from the leaf was recorded after the value was stable. Measurements were carried out at the control values of CO2 concentration (386.1 ± 0.3 µmol mol− 1), leaf temperature (25.2 ± 0.1 °C) and leaf-to-air vapour pressure deficit (VPD 1.5 ± 0.0 kPa) inside of the leaf chamber.
Chl a fluorescence was measured three times using a HandyPEA portable fluorimeter (Hansatech Instruments, Pentney, Norfolk, UK) in July, August and September, 2016. All measurements were carried out in the morning (8:00–10:00). Three plants per treatment were selected and one of the leaves in the intermediate level of the plant canopy was measured after 30 min of dark adaption with leaf clips. Fluorescence transient (FT, that expresses fluorescence induction curve from F0 to Fm in dark-adapted leaves) was analyzed (Strasser et al. 2000). It is well known that FT has a polyphasic behavior (Bussotti et al. 2011). The different phases include step O (20–50 µs), step K (300 µs), step J (2 ms), step I (30 ms) and step P (peak, the highest fluorescence intensity). Here the maximum quantum yield (Fv/Fm) and Chl a fluorescence performance index (PI) were assessed. PI is an integrative index including three independent parameters: the density of active reaction centers on an absorption basis, the quantum yield of primary photochemistry, and the efficiency with which a trapped exciton can move an electron into the electron transport chain further than QA−. PI decreases when the photosynthetic system was damaged by the environmental stress conditions (Appenroth et al. 2001; van Heerden et al. 2003, 2004). The equations for calculating those parameters of Chl a fluorescence are as follows (Strasser et al. 2000):
where RC is the number of active PSII reaction centers, ABS is the quantity of light absorbed by the antenna, VJ is the relative variable fluorescence at 2 ms of the fluorescence rise [denoted as VJ = (F2ms−F0)/(Fm-F0)], and M0 is the slope at the origin of the relative variable fluorescence [denoted as M0 = 4(F300 µs-F0)/(Fm-F0)].
Measurement of Chl content
Chl content was assessed according to Cotrozzi et al. (2017), with minor modifications. Samples (30 mg fresh weight, FW) were collected at early morning at harvest (October 1st) as one intermediate-canopy, fully-expanded leaf per each of the three plants in each treatment, immediately treated by liquid nitrogen and stored at − 80 °C until analysis. They were ground with mortar and pestle, homogenized in 0.3 mL of 100% HPLC-grade methanol and incubated overnight at 4 °C in the dark. High Performance Liquid Chromatography (HPLC; P680 Pump, UVD170U UV–VIS detector, Dionex, Sunnyvale, CA, USA) separation was performed at room temperature with a reverse-phase Dionex column (Acclaim 120, C18, 5 µm particle size, 4.6 mm internal diameter 150 mm length). Chls were eluted at a flow rate of 1 mL min− 1 using 100% solvent A (acetonitrile/methanol, 75/25, v/v) for the first 14 min followed by a 1.5 min linear gradient to 100% solvent B (methanol/ethylacetate, 68/32, v/v), 15 min with 100% solvent B, which was pumped to elute Chl a and b. Chls were detected by their absorbance at 445 nm. Pure authentic standards were used to quantify the pigment contents of each sample. Data were extrapolated by Chromeleon software version 6.60 (Dionex Corporation, Sunnyvale, CA, USA).
Statistical analysis
The study was conducted in a well-replicated split-plot design, with O3 (AA, 1.5 × AA, and 2.0 × AA) as whole-plot factor, and N (N0 and N80) and P (P0 and P80) as split-plot randomized factors. Data were tested for normal distribution by Kolmogorov–Smirnov test. Three-way ANOVA was applied to assess the effect of O3 in plants under different N and P conditions (O3 × N × P). Post-hoc differences were tested by Tukey test (p < 0.05). The relative effect of O3 on each parameter was calculated as [(ValueAA − Value2.0 × AA)/ValueAA]. ValueAA means the value of a certain parameter under AA and Value2.0 × AA means the corresponding value under 2.0 × AA. Simple regression analysis was applied to assess relationships between PN and gs at three levels of O3 in each nutritional treatment. If all regressions at the three O3 levels were statistically significant, we applied an analysis of covariance (ANCOVA) to assess effects of O3 on the relationship between PN and gs.
Results
Gas exchange
From morning to afternoon, PN and gs decreased gradually (Fig. 1). In the morning, elevated O3 significantly decreased both PN and gs relative to AA (PN − 5% in 1.5 × AA and − 26% in 2.0 × AA; gs − 1% in 1.5 × AA and − 23% in 2.0 × AA). Nitrogen addition significantly increased PN relative to N0 (+ 20%). There was a significant interaction between O3 and N on PN. The loss of PN caused by medium O3 exposure (1.5 × AA) was alleviated by N addition, although such an alleviating effect of N was not found in 2.0 × AA. At noon, PN and gs were significantly decreased by O3 (PN − 18% in 1.5 × AA and − 38% in 2.0 × AA; gs − 35% in 1.5 × AA and − 45% in 2.0 × AA) and increased by N80 (PN + 32%; gs + 27%). However, the O3 × N interaction was not significant. PN and gs in the afternoon were not affected by any treatments. The Ci/Ca ratio was decreased by N addition (–5%) in the morning (Fig. 1). Regardless of measurement time of day, a significant interaction was found between O3 and P, indicating that increases of the Ci/Ca ratio due to O3 exposure were limited by P treatments (i.e., P0 treatments: + 6% in the morning, + 6% at noon and + 10% in the afternoon in 2.0 × AA relative to AA; on the other hand, P80 treatments: − 2% in the morning, − 3% at noon and − 7% in the afternoon in 2.0 × AA relative to AA). Dark respiration rate (Rd) was significantly increased by O3 (Fig. 2, + 58% in 1.5 × AA and + 108% in 2.0 × AA). Nitrogen increased Rd by 59% while P did not change it significantly. There was a significant interaction of O3 and N in that the increase of Rd due to O3 exposure was enhanced due to the N treatment.
Daily changes of net photosynthetic rate (PN, µmol m− 2 s− 1), stomatal conductance (gs, mol m− 2 s− 1) and the ratio of leaf-internal to ambient CO2 concentration (Ci/Ca ratio) of poplar Oxford clone under different O3 concentrations (AA, ambient O3 concentration; 1.5 × AA; 2.0 × AA) and subjected to four combinations of nutrient treatment in the soil [two levels of N (0 and 80 kg N ha− 1; N0 and N80) and two levels of P (0 and 80 kg P ha− 1; P0 and P80)]. The bars represent mean ± S.E. (n = 3). Asterisks show the significance of a 3w ANOVA: ***p < 0.001, **p < 0.01, *p < 0.05, ns not significant. Different letters show significant differences among treatments (p < 0.05, Tukey test)
Dark respiration rate (µmol m− 2 s− 1) in Populus maximoviczii Henry × P. berolinensis Dippel (clone Oxford) leaves under free air O3 exposure [applied for five consecutive months: ambient air (AA), 1.5 × and 2.0 × ambient O3 (1.5 × AA and 2.0 × AA)] and subject to four combinations of nutrient treatment in the soil [two levels of N (0 and 80 kg N ha− 1; N0 and N80) and two levels of P (0 and 80 kg P ha− 1; P0 and P80)]. Data are shown as mean ± S.E. (n = 3). Different letters show significant differences among treatments (p < 0.05, Tukey test). Asterisks show the significance of a 3w ANOVA: ***p < 0.001, *p < 0.05
Chl fluorescence
The effect of the combination of O3, N and P treatments on the chl fluorescence parameters of poplar leaves are shown in Fig. 3. At any measurement time, Fv/Fm was decreased by elevated O3 (1.5 × AA − 1% in July, − 1% in August, and − 2% in September; 2.0 × AA − 2% in July, -1% in August, and − 9% in September). The values of Fv/Fm were slightly but statistically significantly increased by N supply in July (+ 1%). Phosphorus addition decreased Fv/Fm in July (–1%) and September (–1%) but increased it in August (+ 1%). We found an interactive effect of O3 × N on Fv/Fm in July, suggesting a mitigation effect of N on the O3 damage to this Chl fluorescence parameter. However, such an effect was not clear in the other months. Ozone-induced loss of Fv/Fm was higher in P enrichment as confirmed by a significant interaction between O3 and P on Fv/Fm in July and August. This suggests that P exacerbated negative effects of O3 on Fv/Fm. We also found an interactive effect of N × P on Fv/Fm in September, confirming that N addition increased the Fv/Fm values at P0 (+ 2%) and decreased them at P80 (–3%). The interaction of the three factors (O3 × N × P) was significant for Fv/Fm in September. This indicates that the O3-caused losses of Fv/Fm were aggravated when adding N and P together.
Monthly values of the maximum quantum yield of primary photochemistry (Fv/Fm) and the performance index (PI) under different O3 concentrations (AA, ambient O3 concentration; 1.5 × AA; 2.0 × AA) and subjected to four combinations of nutrient treatment in the soil [two levels of N (0 and 80 kg N ha− 1; N0 and N80) and two levels of P (0 and 80 kg P ha− 1; P0 and P80)]. The bars represent mean ± S.E. (n = 3). Different letters show significant differences among treatments (p < 0.05, Tukey test). Asterisks show the significance of a 3w ANOVA: ***p < 0.001, **p < 0.01, *p < 0.05
Chl a fluorescence performance index (PI) was reduced by O3 in July (–26% in 1.5 × AA, − 23% in 2.0 × AA) and September (–26% in 1.5 × AA, − 62% in 2.0 × AA), although increases of PI were found in elevated O3 in August (+ 15% in 1.5 × AA, + 27% in 2.0 × AA) (Fig. 3). Nitrogen supply significantly increased PI in August (+ 23%) and September (+ 30%). Phosphorus addition decreased PI in September (–20%). Similarly to Fv/Fm, a mitigation effect of N on O3-induced losses of PI was found in July, as confirmed by the statistical significance of O3 × N. The analysis shows that there was a significant interaction on PI between N and P in September. Nitrogen increased PI values at low P (+ 69%) and decreased them at high P treatments (–6%).
Total Chl content
The three-way ANOVA test of the total Chl content revealed that all the effects and their interactions were significant (Fig. 4). Compared to AA, O3 per se slightly decreased the total Chl content (–10% and − 14% in 1.5 × AA and 2.0 × AA). Nitrogen addition significantly increased the total Chl content when all N80 treatments were compared to all N0 ones. The relative rise was higher in N80P0 than in N0P0 under 2.0 × AA conditions (+ 63%). However, total Chls were not affected by N enrichment under 1.5 × AA (except under N80P80). Compared to P0, P addition decreased the total Chl content, independently of O3 levels and N addition (except in N80P80 and N0P80 under AA and 2.0 × AA): the relative loss was highest in N80P80 than in N80P0 under 1.5 × AA conditions (–48%).
Total Chl content (µmol g DW− 1) in Populus maximoviczii Henry × P. berolinensis Dippel (clone Oxford) leaves under free air O3 exposure [applied for five consecutive months: ambient air (AA), 1.5 × and 2.0 × ambient O3 (1.5 × AA and 2.0 × AA)] and subject to four combinations of nutrient treatment in the soil [two levels of N (0 and 80 kg N ha− 1; N0 and N80) and two levels of P (0 and 80 kg P ha− 1; P0 and P80)]. Data are shown as mean ± S.E. (n = 3). Different letters show significant differences among treatments (p < 0.05, Tukey test). Asterisks show the significance of a 3w ANOVA: ***p < 0.001
Discussion
The present study showed that N addition significantly increased PN of poplars in the morning (Fig. 1), which is consistent with other studies (e.g., Yamaguchi et al. 2007). In addition, the N supply (80 kg ha− 1 year− 1) reduced the relative loss of PN induced by 1.5 × AA O3 level, although such a mitigation was not observed in the higher level of O3 concentration (2.0 × AA). Nitrogen addition may stimulate leaf turnover forming new leaves with elevated photosynthesis, which might counteract the decrease of PN induced by O3 in damaged older leaves (Maurer and Matyssek 1997; Pell et al. 1995). However, such a response did not fully compensate the loss of carbon gain due to O3 exposure. We also found that Rd was increased by O3, as previously reported in Japanese and European beeches (Hoshika et al. 2013; Kitao et al. 2009). It has been postulated that the increase in Rd under elevated O3 may be attributed to demand for O3 detoxification and repair of damaged tissues (Matyssek and Sandermann 2003). Interestingly, O3-induced increase of Rd was more evident with N addition. This suggests that O3 stress is more severe under high N availability. Nitrogen addition may thus lower the O3 tolerance of Oxford poplar clone. The respiratory carbon loss can be considered a major cause for the reduction of carbon gain due to elevated O3 (Watanabe et al. 2014a). In our previous paper (Zhang et al. 2018), we found that O3-induced decrease of poplar biomass was greater at high N supply. The increased respiratory carbon loss by O3 × N treatments may result in a further decrease in net carbon gain under O3.
Stomatal conductance is generally correlated with photosynthetic rate to keep a nearly constant ratio of Ci/Ca (Larcher 2003). However, in particular in P0 treatments, elevated O3 caused a significant increase of the Ci/Ca ratio, which explains a relative decrease in stomatal limitation to photosynthesis and an increase in non-stomatal limitations such as biochemical limitations (Farage and Long 1995; Watanabe et al. 2014b). Such an increase of the Ci/Ca ratio after O3 exposure was probably a result of a decoupling of gs from PN, i.e., gs remained relatively high (0.3–0.4 mol m− 2 s− 1) even though a significant decrease of PN was found in 2.0 × AA (Fig. S1). The decoupling of gs from PN may be attributed to an impairment of stomatal control after O3 exposure (i.e., stomatal sluggishness, Paoletti 2005), which results in decreases in the intrinsic water use efficiency (iWUE, denoted as the ratio of PN to gs). However, such a response was not found in P80 treatments. With P addition, elevated O3 exhibited declining PN and gs, while maintaining the Ci/Ca ratio as in the control. Phosphorus plays an important role in energy production as components of ATP, which acts as an energy currency of guard cells for stomatal movement (Marten et al. 2007; Suetsugu et al. 2014), involving in controlling key enzyme reactions and in the regulation of metabolic pathways (Larcher 2003). Phosphorus may therefore improve the O3-induced reduction of iWUE.
Chl a fluorescence is a useful tool to unveil the change of the excitation energy in PSII and estimate the status of PSII (Kalaji et al. 2014). Ozone caused a reduction in Fv/Fm and PI throughout most of the experimental period (Fig. 3, S2), although a temporal increase of PI of poplar leaves under elevated O3 was found in August. This decrease of Fv/Fm may relate to photoinhibition (Bussotti et al. 2011; Zhang et al. 2010). The reduction of PI indicates the development of O3 damage with the increase in energy dissipation through thermal losses (Bussotti et al. 2011). The temporarily higher PI under O3 stress in August indicated an enhanced efficiency of electron trapping and transport which might be related to the repair processes (Bussotti et al. 2011). Nitrogen addition ameliorated the O3-induced decrease of Fv/Fm and PI in July as previously observed in aspen (Häikiö et al. 2007) and manna ash (Fusaro et al. 2017). Nitrogen-fertilized plants are considered more tolerant to photoinhibition due to their higher photosynthetic energy consumption (e.g. Ferrar and Osmond 1986), although Kato et al. (2002) suggested a similar susceptibility to photoinhibition between high and low N-fertilized Chenopodium album plants because the low N-fertilized plants may dissipate large excess energy by non-photochemical quenching to compensate for the lower photosynthetic energy utilization. At the end of the growing season, however, an O3-induced decrease of Fv/Fm was found regardless of N availability, suggesting a significant decrease of photosynthetic efficiency. On the other hand, the addition of P exacerbated the loss of Fv/Fm in July and August, which indicates a lower capacity of protection against photoinhibition. Asada (1999) pointed out a contribution of energy dissipation by the water–water cycle to the protection against photoinhibition. Phosphorus enrichment may decrease the content of ascorbate peroxidase (Desai et al. 2014), which is essential for the water–water cycle (Awad et al. 2015).
The effects of O3 on total Chl content were changed by N and/or P treatments. The nutrient-rich conditions resulted in even higher Chl concentration in 2.0 × AA relative to AA. Similarly, Han et al. (2009) reported that fertilized Platanus occidentalis trees formed leaves with 1.5 times higher Chl content after O3 exposure, suggesting an overcompensation against O3 stress (Kitao et al. 2015). Such an overcompensation may be interpreted as a hormetic response (Sugai et al. 2018). The result, however, indicated that this increase of Chl content did not eventually protect the electron transport activity in PSII from O3 damage.
In conclusion, as we postulated, the availability of N and P influenced the photosynthetic responses to O3. Our results indicate that P ameliorated the O3-induced reduction of iWUE while it aggravated the O3-induced damage to Chl a fluorescence. N could alleviate negative effects of O3 on Chl a fluorescence parameters in early summer. Although N addition partly mitigated O3-induced loss of PN (only in 1.5 × AA), such a mitigation effect was not observed in the higher O3 level (2.0 × AA). In addition to the decrease of PN, N exacerbated an increase of Rd due to O3 exposure, which results in further reductions in net carbon gain. Overall, O3 decreased the photosynthetic capacity of poplars regardless of the different nutritional conditions. Further studies on the responses of the antioxidant metabolism to O3, N and P may help reconcile the contrasting effects on plant O3 sensitivity observed so far.
References
Appenroth K-J, Stöckel J, Srivastava A, Strasser RJ (2001) Multiple effects of chromate on the photosynthetic apparatus of Spirodela polyrhiza as probed by OJIP Chl fluorescence measurements. Environ Pollut 115:49–64
Arevalo CBM, Bhatti JS, Chang SX, Sidders D (2011) Land use change effects on ecosystem carbon balance: from agricultural to hybrid poplar plantation. Agric Ecosys Environ 141:342–349
Asada K (1999) The water–water cycle in chloroplasts: scavenging of active oxygens and dissipation of excess photons. Ann Rev Plant Physiol Plant Mol Biol 50:601–639
Awad J, Stotz HU, Fekete A, Krischke M, Engert C, Havaux M, Berger S, Mueller MJ (2015) 2-cysteine peroxiredoxins and thylakoid ascorbate peroxidase create a water–water cycle that is essential to protect the photosynthetic apparatus under high light stress conditions. Plant Physiol 167:1592–1608
Bowen HJM (1979) Environmental chemistry of the elements. Academic Press, London
Braun S, Achermann B, De Marco A, Pleijel H, Karlsson PE, Rihm B, Schindler C, Paoletti E (2017) Epidemiological analysis of ozone and nitrogen impacts on vegetation—critical evaluation and recommendations. Sci Tot Environ 603–604:785–792
Bryant JP, Chapin FS III, Klein DR (1983) Carbon/nutrient balance of boreal plants in relation to vertebrate herbivory. Oikos 40:357–368
Bussotti F, Desotgiu R, Cascio C, Pollastrini M, Gravano E, Gerosa G, Marzuoli R, Nali C, Lorenzini G, Salvatori E, Manes F, Schaub M, Strasser RJ (2011) Ozone stress in woody plants assessed with chlorophyll a fluorescence. A critical reassessment of existing data. Environ Exp Bot 73:19–30
Christersson L (2010) Wood production potential in poplar plantations in Sweden. Biomass Bioener 34:1289–1299
Cooper OR, Parrish DD, Ziemke J, Balashov NV, Cupeiro M, Galbally IE, Gilge S, Horowitz L, Jensen NR, Lamarque J-F, Naik V, Oltmans SJ, Schwab J, Shindell DT, Thompson AM, Thouret V, Wang Y, Zbinden RM (2014) Global distribution and trends of tropospheric ozone: anobservation-based review. Elem Sci Anth 2:000029
Cotrozzi L, Remorini D, Pellegrini E, Guidi L, Nali C, Lorenzini G, Massai R, Landi M (2017) Living in a Mediterranean city in 2050: broadleaf or evergreen “citizens”? Environ Sci Pollut Res. https://doi.org/10.1007/s11356-017-9316-7
Crutzen PJ (1970) The influence of nitrogen oxides on the atmospheric ozone content. Q J Roy Meteorol Soc 96:320–325
Desai S, Nak D, Cumming JR (2014) The influence of phosphorus availability and Laccaria bicolor symbiosis on phosphate acquisition, antioxidant enzyme activity, and rhizospheric carbon flux in Populus tremuloides. Mycorrhiza 24:369–382
Farage PK, Long SP (1995) An in vivo analysis of photosynthesis during short-term O3 exposure in three contrasting species. Photosyn Res 43:11–18
Feng Z, Niu J, Zhang W, Wang X, Yao F, Tian Y (2011) Effects of ozone exposure on sub-tropical evergreen Cinnamomum camphora seedlings grown in different nitrogen loads. Trees 25:617–625
Fenn ME, Baron JS, Allen EB, Rueth HM, Nydick KR, Geiser L, Bowman WD, Sickman JO, Meixner T, Johnson DW, Neitlich P (2003) Ecological effects of nitrogen deposition in the western United States. Bioscience 53:391–403
Ferrar PJ, Osmond CB (1986) Nitrogen supply as a factor influencing photoinhibition and photosynthetic acclimation after transfer of shade-grown Solanum dulcamara to bright light. Planta 168:563–570
Fusaro L, Palma A, Salvatori E, Basile A, Maresca V, Karam EA, Manes F (2017) Functional indicators of response mechanisms to nitrogen deposition, ozone, and their interaction in two Mediterranean tree species. PloS one 12:e0185836
Häikiö E, Freiwald V, Silfver T, Beuker E, Holopainen T, Oksanen E (2007) Impacts of elevated ozone and nitrogen on growth and photosynthesis of European aspen (Populus tremula) and hybrid aspen (P. tremula × Populus tremuloides) clones. Can J For Res 37:2326–2336
Han S-H, Kim D-H, Lee J-C, Kim P-G (2009) Effects of fertilization on physiological parameters in American scamore (Platanus occidentalis) during ozone stress and recovery phase. J Ecol Field Biol 32:149–158
Harmens H, Hayes F, Sharps K, Mills G, Calatayud V (2017) Leaf traits and photosynthetic responses of Betula pendula saplings to a range of ground-level ozone concentrations at a range of nitrogen loads. J Plant Physiol 211:42–52
Hoosbeek MR, Lukac M, van Dam D, Godbold DL, Velthorst EJ, Biondi FA, Peressotti A, Cortufo MF, de Angelis P, Scarascia-Mugnozza G (2004) More new carbon in the mineral soil of a poplar plantation under free air carbon enrichment (POPFACE): cause of increased priming effect? Glob Biogeochem Cycles 18:GB1040. https://doi.org/10.1029/2003GB002127
Hoshika Y, Watanabe M, Inada N, Koike T (2013) Model-based analysis of avoidance of ozone stress by stomatal closure in Siebold’s beech (Fagus crenata). Ann Bot 112:1149–1158
Hoshika Y, Carrari E, Zhang L, Carriero G, Pignatelli S, Fasano G, Materassi A, Paoletti E (2018) Testing a ratio of photosynthesis to O3 uptake as an index for assessing O3-induced foliar visible injury in poplar trees. Environ Sci Pollut Res 25:8113–8124
Kalaji HM, Schansker G, Ladle RJ, Goltsev V, Bosa K, Allakhverdiev SI, Brestic M, Bussotti F, Calatayud A, Dąbrowski P, Elsheery NI, Ferroni L, Guidi L, Hogewoning SW, Jajoo A, Misra AN, Nebauer SG, Pancaldi S, Penella C, Poli DB, Pollastrini M, Romanowska-Duda ZB, Rutkowska B, Serôdio J, Suresh K, Szulc W, Tambussi E, Yanniccari M, Zivcak M (2014) Frequently asked questions about chlorophyll fluorescence: practical issues. Photosyn Res 122:121–158
Kato MC, Hikosaka K, Hirose T (2002) Photoinactivation and recovery of photosystem II in Chenopodium album leaves grown at different levels of irradiance and nitrogen-availability. Funct Plant Biol 29:787–795
Kitao M, Löw M, Heerdt C, Grams TEE, Häberle K-H, Matyssek R (2009) Effects of chronic elevated ozone exposure on gas exchange responses of adult beech trees (Fagus sylvatica) as related to the within-canopy light gradient. Environ Pollut 157:537–544
Kitao M, Kamatsu M, Yazaki K, Kitaoka S, Tobita H (2015) Growth overcompensation against O3 exposure in two Japanese oak species, Quercus mongolica var. crispula and Quercus serrata, grown under elevated CO2. Environ Pollut 206:133–141
Koerselman W, Meuleman AFM (1996) The vegetation N:P ratio: a new tool to detect the nature of nutrient limitation. J Appl Ecol 33:1441–1450
Koricheva J (2002) The carbon-nutrient balance hypothesis is dead; long live the carbon-nutrient balance hypothesis? Oikos 98:537–539
Larcher W (2003) Physiological plant ecology, 4. Springer-Verlag, New York
Lewis JD, Strain BR (1996) The role of mycorrhizas in the response of Pinus taeda seedlings to elevated CO2. New Phytol. 133:431–443
Marschner H (2011) Marschner’s mineral nutrition of higher plants, 3rd edn. Academic Press, Cambridge
Marten H, Konrad KR, Dietrich P, Roelfsema RG, Hedrich R (2007) Ca2+ -dependent and -independent abscisic acid activation of plasma membrane anion channels in guard cells of Nicotiana tabacum. Plant Physiol 143:28–37
Matyssek R, Sandermann H (2003) Impact of ozone on trees: an ecophysiological perspective. In: Esser K, Lüttge U, Beyschlag W, Hellwig F (eds) An ecophysiological perspective. Progress in Botany, vol 64. Springer-Verlag, Berlin, pp 349–404
Matyssek R, Clarke N, Cudlín P, Mikkelsen TN, Tuovinen JP, Wieser G, Paoletti E (2013) Climate change, airpollution and global challenges: understanding and perspectives from forest research. In: Krupa SV (ed) Developments in environmental science. Elsevier, Amsterdam, p 622
Maurer S, Matyssek R (1997) Nutrition and the ozone sensitivity of birch (Betula pendula). II. Carbon balance, water-use efficiency and nutritional status of the whole plant. Trees 12:11–20
Maurer S, Matyssek R, Günthardt-Goerg MS, Landolt W, Einig W (1997) Nutrition and the ozone sensitivity of birch (Betula pendula). I. Responses at the leaf level. Trees 12:1–10
Mills G, Pleijel H, Malley CS, Sinha B, Cooper O, Schultz M, Neufeld HS, Simpson D, Sharps K, Feng Z, Gerosa G, Harmens H, Kobayashi K, Saxena P, Paoletti E, Sinha V, Xu X (2018) Tropospheric ozone assessment report: present day tropospheric ozone distribution and trends relevant to vegetation. Elem Sci Anth 6:47. https://doi.org/10.1525/elementa.302
Ollinger SV, Aber JD, Reich PB, Freuder RJ (2002) Interactive effects of nitrogen deposition, tropospheric ozone, elevated CO2 and land use history on the carbon dynamics of northern hardwood forests. Glob Chan Biol 8:545–562
Ormrod DP, Adedipe NO, Hofstra G (1973) Ozone effects on growth of radish plants as influenced by nitrogen and phosphorus nutrition and by temperature. Plant Soil 39:437–439
Paoletti E (2005) Ozone slows stomatal response to light and leaf wounding in a Mediterranean evergreen broadleaf, Arbutus unedo. Environ Pollut 134:439–445
Paoletti E, De Marco A, Beddows DCS, Harrison RM, Manning WJ (2014) Ozone levels in European and USA cities are increasing more than at rural sites, while peak values are decreasing. Environ Pollut 192:295–299
Paoletti E, Materassi A, Fasano G, Hoshika Y, Carriero G, Silaghi D, Badea O (2017) A new-generation 3D ozone FACE (free air controlled exposure). Sci Total Environ 575:1407–1414
Pell EJ, Sinn JP, Johansen CJ (1995) Nitrogen supply as a limiting factor determining the sensitivity of Populus tremuloides Michx. to ozone stress. New Phytol 130:437–446
Peng Y, Chen G, Chen G, Li S, Peng T, Qiu X, Luo J, Yang S, Hu T, Hu H, Xu Z, Liu L, Tang Y, Tu L (2017) Soil biochemical responses to nitrogen addition in a secondary evergreen broad-leaved forest ecosystem. Sci Rep 7:2783. https://doi.org/10.1038/s41598-017-03044-w
Peñuelas J, Sardans J, Rivas-Ubach A, Janssens IA (2012) The human-induced imbalance between C, N and P in Earth’s life system. Glob Chan Biol 18:3–6
Schulze ED (1989) Air pollution and forest decline in a spruce (Picea abies) forest. Science 244:776–783
Sicard P, De Marco A, Troussier F, Renou C, Vas N, Paoletti E (2013) Decrease in surface ozone concentrations at Mediterranean remote sites and increase in the cities. Atmos Environ 79:705–715
Stevenson FJ, Cole MA (1999) Cycles of soil (carbon, nitrogen phosphorus sulfur, micronutrients). John Wiley and Sons Publishers, Hoboken
Strasser RJ, Srivastava A. Tsimilli-Michael M (2000) The fluorescence transient as a tool to characterize and screen photosynthetic samples. In: Yunus M, Pathre U, Mohanty P (eds) Probing photosynthesis: mechanisms, regulation and adaptation. Taylor and Francis, London, pp 445–483
Strasser RJ, Tsimilli-Michael M, Qiang S, Goltsev V (2010) Simultaneous in vivo recording of prompt and delayed fluorescence and 820-nm reflection changes during drying and after rehydration of the resurrection plant Haberlea rhodopensis. Biochim Biophys Acta 1797:1313–1326
Suetsugu N, Takami T, Ebisu Y, Watanabe H, Iiboshi C, Doi M, Shimazaki K (2014) Guard cell chloroplasts are essential for blue light-dependent stomatal opening in Arabidopsis. Plos one 9:e108374
Sutinen S, Wallin G, Karlsson PE, Skärby L, Selldén G (1998) Cell ultrastructure of needles from saplings of Norway spruce, Picea abies (L) Karst., exposed to ozone and low phosphorus supply in open-top chambers. Chemosphere 36:691–696
Sugai T, Kam D-G, Agathokleous E, Watanabe M, Kita K, Koike T (2018) Growth and photosynthetic response of two larches exposed to O3 mixing ratios ranging from preindustrial to near future. Photosynthetica 56:901–910
Thomas RB, Lewis JD, Strain BR (1994) Effects of leaf nutrient status on photosynthetic capacity in loblolly pine (Pinus taeda L.) seedlings grown in elevated atmospheric CO2. Tree Physiol 14:947–960
Tissue DT, Lewis JD (2010) Photosynthetic responses of cottonwood seedlings grown in glacial through atmospheric [CO2] vary with phosphorus supply. Tree Physiol 30:1361–1372
Tjoelker MG, Luxmoore RJ (1991) Soil nitrogen and chronic ozone stress influence physiology, growth and nutrient status of Pinus taeda L. and Liriodendron tulipifera L. seedlings. New Phytol 119:69–81
Utriainen J, Holopainen T (2001) Influence of nitrogen and phosphorous availability and ozone stress on Norway spruce seedlings. Tree Physiol 21:447–456
Utriainen J, Holopainen T (2002) Responses of Pinus sylvestris and Picea abies seedlings to limited phosphorus fertilization and treatment with elevated ozone concentrations. Scand J For Res 17:501–510
van Heerden PDR, Tsimilli-Michael M, Krüger GHJ, Strasser RJ (2003) Dark chilling effects on soybean genotypes during vegetable development: parallel studies of CO2 assimilation, chlorophyll a fluorescence kinetics O–J–I–P and nitrogen fixation. Physiol Plant 117:476–491
van Heerden PDR, Strasser RJ, Krüger GHJ (2004) Reduction of dark chilling stress in N2-fixing soybean by nitrate as indicated by chlorophyll a fluorescence kinetics. Physiol Plant 121:239–249
Vingarzan R (2004) A review of surface ozone background levels and trends. Atmos Environ 38:3431–3442
Warren CR (2011) How does P affect photosynthesis and metabolite profiles of Eucalyptus globulus? Tree Physiol 31:727–739
Watanabe M, Yamaguchi M, Iwasaki M, Matsuo N, Naba J, Tabe C, Matsumura H, Kohno Y, Izuta T (2006) Effects of ozone and/ or nitrogen load on the growth of Larix kaempferi, Pinus densiflora and Cryptomeria japonica seedlings. J Jpn Soc Atmos Environ 41:320–334
Watanabe M, Hoshika Y, Inada N, Koike T (2014a) Canopy carbon budget of siebold’s beech (Fagus crenata) sapling under free air ozone exposure. Environ Pollut 184:682–689
Watanabe M, Hoshika Y, Koike T (2014b) Photosynthetic responses of monarch birch seedlings to differing timings of free air ozone fumigation. J Plant Res 127:339–345
Whitfield CP, Davison AW, Ashenden TW (1998) The effects of nutrient limitation on the response of Plantago major to ozone. New Phytol 140:219–230
Wilkinson S, Davies W (2010) Drought, ozone, ABA and ethylene: new insights from cell to plant community. Plant Cell Environ 33:510–525
Wooliver RC, Marion ZH, Peterson CR, Potts BM, Senior JK, Bailey JK, Schweitzer JA (2017) Phylogeny is a powerful tool for predicting plant biomass responses to nitrogen enrichment. Ecology 98:2120–2132
Yamaguchi M, Watanabe M, Iwasaki M, Tabe C, Matsumura H, Kohno Y, Izuta T (2007) Growth and photosynthetic responses of Fagus crenata seedlings to O3 under different nitrogen loads. Trees 21:707–718
Zhang L, Xu H, Yang JC, Li WD, Jiang GM, Li YG (2010) Photosynthetic characteristics of diploid honeysuckle (Lonicera japonica Thunb.) and its autotetraploid cultivar subjected to elevated ozone exposure. Photosynthetica 46:87–95
Zhang L, Hoshika Y, Carrari E, Badea O, Paoletti E (2018) Ozone risk assessment is affected by nutrient availability: evidence from a simulation experiment under free air controlled exposure (FACE). Environ Pollut 238:812–822
Acknowledgements
This work was financially supported by National Natural Science Foundation of China (31711530648), University Nursing Program for Young Scholar With Creative Talents in Heilongjiang Province (UNPYSCT-2017023), the Fondazione Cassa di Risparmio di Firenze (2013/7956) and the LIFE15 ENV/IT/000183 project MOTTLES. We would like to thank Alessandro Materassi, Gianni Fasano, Moreno Lazzara, Lorenzo Bussotti, and Isaac Tasap for their support during the field work. We thank Matthew Haworth for the English proofreading.
Author information
Authors and Affiliations
Corresponding author
Rights and permissions
About this article
Cite this article
Zhang, L., Hoshika, Y., Carrari, E. et al. Effects of nitrogen and phosphorus imbalance on photosynthetic traits of poplar Oxford clone under ozone pollution. J Plant Res 131, 915–924 (2018). https://doi.org/10.1007/s10265-018-1071-4
Received:
Accepted:
Published:
Issue Date:
DOI: https://doi.org/10.1007/s10265-018-1071-4