Abstract
Natural killer (NK) cells are cytotoxic lymphocytes that play a major role in the innate immune system. NK cells exhibit potent cytotoxic activity against cancer cells and virally infected cells without antigen priming. These unique cytotoxic properties make NK cells a promising therapeutic against cancer. Limitations of NK cell therapy include deficiencies in high clinical efficacy often due to a need for a high NK cell to target cell ratio to achieve effective killing. In order to address the suboptimal efficacy of current adoptive NK cell therapy, a high throughput screen (HTS) was designed and performed to identify drug-like compounds that increase NK cytotoxic activity against tumor cells without affecting the normal cells. This screen was performed in a 384-well plate format utilizing an expanded primary NK cell product and ovarian cancer cells as a target cell (TC) line. Of the 8000 diverse small molecules screened, 16 hits were identified (0.2% hit rate) based on both a robust Z (RZ) score < -3 and a greater than 10% increase in NK cell killing. A validation screen had a confirmation rate of 70%. Select compounds were further validated and characterized by additional cytotoxicity assays including activity against multiple blood cancer and solid tumor cell lines, with no effect on primary human T cells. This work demonstrates that high-throughput screening can be reliably used to identify compounds that increase NK tumoricidal activity in vitro that can be further investigated and translated for potential clinical application. Précis: Our work led to the identification of promising compound that potently increases NK cell-mediated killing of a variety of different cancer cells, but no impact on the killing of normal cells. This compound demonstrates the utility of this assay.
Similar content being viewed by others
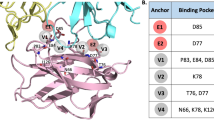
Avoid common mistakes on your manuscript.
Introduction
NK cells are cytotoxic lymphocytes that play a central role in the innate immune system and provide cell-mediated immunity against viruses and malignantly transformed cells [1]. NK cells are able to specifically target virally infected cancer cells due to a balance of signals emanating from large families of inhibitory and activating receptors on their surface [2][3]. The balance of activating versus inhibitory signals is thought to determine if NK cells lyse target cells. A net activating stimulus leads to the release of lytic granules containing perforin and granzyme which can induce apoptosis in the engaged target cell (TC)[4].
Due to the unique capacity of NK cells to recognize and directly lyse TCs, they are being investigated in the context of adoptive cell therapy for cancer [5]. NK cell immunotherapy has shown particular promise in treating hematologic malignancies such as acute myeloid leukemia (AML) and B cell malignancies [1]. For example, in a clinical trial of NK cell immunotherapy involving 11 patients with B-cell malignancies, seven patients achieved full remission (four with lymphoma and three with chronic lymphocytic leukemia), and one patient had a partial remission [6]. NK cell therapy has also proven to be effective against other malignancies such as diffuse large B cell lymphoma, multiple myeloma, prostate, ovarian, and cervical cancer [5, 7]–[11]. NK cell therapy offers many potential advantages over other immune cell therapy types, such as T cell immunotherapy, including the ability to target cancer cells without the necessity for tumor antigen specific recognition and the ability to utilize allogeneic donors without inducing graft versus host disease [12]. In particular, while HLA matching is essential to prevent graft versus host disease with T cell therapies, clinical studies to date suggest that NK cells, in contrast, do not induce graft versus host disease, and therefore, universal donor NK products may be feasible [13].
Our study was directed against ovarian cancer cells. Ovarian cancer is the deadliest gynecological cancer [14]. Previous reports found that ovarian cancer is sensitive to NK lysis, making it a target of current clinical NK therapy studies [15, 16]. While there is some evidence of clinical feasibility in the treatment of ovarian tumors with adoptive NK cell transfer, improvements in NK activity against this malignancy are important to enhance clinical efficacy [16].
Despite the promise of NK cell therapy for cancer, there are some limitations that have hindered the field. One major shortcoming is the challenge to manufacture large doses of NK cells to support an off-the-shelf universal donor product. This has been partially overcome by ex vivo expansion platforms that impair NK cell senescence and permit robust and long-term expansion of clinical grade NK cells [17, 18]. While these expanded NK cells demonstrate increased cytotoxic activity as compared to non-expanded cells and show promise in clinical testing, it is likely that further enhancements in cytotoxic activity will be necessary to achieve marked clinical efficacy [13, 19]. Although patients receiving NK cell therapy have been shown to go into remission, their cancers often relapse, which may be attributable to the short lifespan, insufficient cytotoxic activity, and limited number of NK cells in many adoptive NK cell studies.
Despite the challenges, the potential of NK cell therapy against cancer supports the identification of novel strategies to enhance NK cell cytotoxic activity toward cancer cells. Recently, strategies to modulate the immune system, most commonly T cells, have become more prevalent in cancer therapy. For example, the identification of immune checkpoint blockade has stirred up interest in identifying checkpoint inhibitors, including small molecule inhibitors, particularly against PD1 and CTLA-4[20]. In the case of NK cells, investigations are underway to assess the potential of targeting checkpoint molecules including LAG-3, TIM-3 and TIGIT largely through blocking antibodies [21]. In addition, studies have suggested that blockade of the immunosuppressive cytokine TGFβ by small molecules or antibodies may enhance NK cell activity [22]. A small number of other small molecules have been described that can impact NK cell function, though none that exhibit any known significant clinical efficacy to date. For example, dasatinib, a kinase inhibitor commonly used to treat chronic myeloid leukemia, has been reported to enhance NK cell activity against leukemia cells through unclear mechanisms [23]. Small molecule GSK3 inhibitors have also been found to enhance NK cell cytotoxic activity against cancer cells through enhancing conjugate formation with tumor cells [24].
As the mechanisms and pathways through which NK cells regulate their cytotoxic activity are not fully characterized, we developed an activity-based screen to identify novel small molecules that can enhance NK cell cytotoxic activity. To identify small molecule regulators of NK activity, we chose a high throughput screening (HTS) system because it allows for rapid testing of a large number of drug-like compounds. HTS systems overcome the limited assay capacity for in vitro studies and allow the rapid ability to test hundreds of thousands of compounds with relative ease and cost-effectiveness [25]. In particular, we developed and implemented a cell-based HTS assay using a clinical NK cell product to enable the identification of compounds that may be useful both as tool compounds to better understand NK cell cytotoxicity as well as initial hits that can be developed into clinically useful NK cell activating agents.
Methods
Cell culture
NK cells were isolated from healthy donor peripheral blood acquired through the Hematopoietic Biorepository and Cellular Therapy Core at Case Western Reserve University. NK cell isolation and expansion were performed as previously described [18]. Briefly, Peripheral blood mononuclear cells were first isolated from blood using a ficoll-paque (GE healthcare) density gradient centrifugation. Next, T cells were depleted using CD3 magnetic beads (Miltenyi Biotec). The isolated cells were then cultured with irradiated NKF (mbIL-21 expressing OCI-AML3) feeder cells at a feeder to NK ratio of 5:1 with 100U/mL IL-2 and RPMI 1640-L-glutamine supplemented with 10% serum, 1% penicillin/streptomycin, and 0.1% ciprofloxacin. Cells were expanded for 2.5 weeks with weekly addition of irradiated NKF cells.
NKF feeder cells, Ovcar3, RAJI, HCT116, and HT29 cells (ATCC) were cultured in RPMI 1640 with 2 mM L-glutamine supplemented with 10% serum, 1% penicillin/streptomycin, and 0.1% ciprofloxacin. All cells were incubated at 37 °C and 5% CO2.
Compound library
A small-molecule library, composed of 8000 compounds from Chemical Diversity Labs and ChemBridge Corporation, was assembled as a plate-based, diversity subset of the larger U. T. Southwestern Chemical Library. All compounds were dissolved in DMSO to a stock concentration of 5 mM. The final DMSO concentration did not exceed 0.1% in the screening assay or during hit validation, and the negative and positive control samples contained the same vehicle percentage.
High-throughput small molecules screening
For high-throughput screening, 384-well plates (Greiner Bio-One) containing a final test concentration of 5 μM of each chemical in columns 3–22 and vehicle (DMSO, 0.1%) in other control wells (columns 1, 2, 23) were prepared using an Echo 555 acoustic liquid handler (Labcyte, Inc., San Jose, CA). A total of 50 μL of media with IL-2 activated NK cells were dispensed into each well of the plates using an automated dispenser (BioTek MultiFlo liquid handler) and incubated 16 h. After an overnight incubation at 37 °C and 5% CO2, 25 μL of media with Calcein-AM labeled Ovcar3 TC were added to all wells at an NK:TC ratio of 1.5:1, followed by a 2-h incubation at 37 ºC in 5% CO2 incubator. In each plate, the following controls were included: 1) a neutral control (DMSO only) of NK and TC at a 1.5:1 ratio (columns 2 and 23); 2) an untreated control of only TC (column 24); and 3) a positive control of NK and TC at a 7.5:1 ratio (column 1). Killing of the tumor cells by the immune effector cells was monitored and recorded by performing a Calcein-AM release cell imaging assay.
Cell imaging, image analysis, and cell viability calculation
Imaging was performed by an IN Cell Analyzer 6000 (Cytiva, Inc.) high content imaging system using a 488 laser line and 20X objective with the assay plate placed on a heated stage during image collection. After images were collected, image analysis was performed using the CellProfiler software (v3.1.8; Broad Institute). Intact TC were identified based on the Calcein-AM fluorescence intensity. Four non-consecutive fields per well were imaged and analyzed. The average number of live and total TC was determined for each well. The viability data were analyzed using Genedata’s Screener software suite (v15) as previously described [26]. Robust Z scores (RZ scores) were assigned to all library compounds [27].
LC–MS and HPLC
Compound 2 was purchased from ChemBridge to perform biological studies, and purity was measured to be > 95% (Supplementary Fig. 2a-c). Reverse phase column was used for both measurements. Water and acetonitrile were used as solvents. LC–MS (Shimadzu LCMS 2020) utilized 0.1% formic acid additive (Supplementary Fig. 2a and b). 0.05% ammonia hydroxide additive was used for HPLC (Agilent 1200) (Supplementary Fig. 2c).
Luciferase-based Cytotoxicity Assay.
Compound treated NK cells were co-cultured with Ovcar3 cells stably expressing a luciferase plasmid (pLenti CMV puro LUC, Addgene) in white bottom 96-well plates for 2 h. After incubation, luciferin substrate (GoldBio, 16.6 μg /mL) was added to the plate for 2 min at room temperature. Luminescence was then measured using a Spectramax Gemini dual-scanning microplate spectrofluorimeter (Molecular Devices). The results of the co-culture were normalized to TC alone wells to calculate % cell death.
Flow cytometry phenotyping
NK cell immunophenotyping was performed using the following antibodies: perforin (BD, PE), granzyme (BD, FITC), IFNγ (BD, PE), TNFα (BD, FITC), CD56 (BD, APC), CD3 (BD, BV421). For intracellular staining, treated NK cells were co-cultured with Ovcar3 cells for 4 h at an ET of 0.5–1 in the presence of monensin (BD GolgiStop, 4 μL/6 mL cell culture). Samples were fixed and permeabilized using the Foxp3/Transcription Factor Staining Buffer Set (eBioscience). Flow cytometry was performed using the Attune Nxt cytometer (ThermoFisher). Analysis was done using FlowJo (version 10.7.1, BD).
Statistical analysis
Nonparametric two-tailed unpaired t tests were performed to determine statistical significance between 2 groups. Nonparametric one-way ANOVA was used to calculate significance between groups of 3 or more. *P < 0.05, **P < 0.01, ***P < 0.001, ****P < 0.0001.
Regulatory approvals
The work using human primary NK cells was performed according to the human subjects guidelines at University Hospitals Cleveland Medical Center and approved by their Institutional Review Board. Informed consent was obtained from all subjects.
Results
Development of high-throughput screen
A high-throughput screen to identify novel small molecules that enhance NK cell cytotoxic activity was developed and optimized in a 384-well plate format (Fig. 1a and b). As a major purpose of this screen is to develop strategies to enhance clinical adoptive NK cell therapy, expanded primary human NK cells served as the basis of the screen. Specifically, NK cells expanded using NKF feeder cells that are currently being tested as a therapeutic in relapsed/refractory cancer patients were utilized [28]. NK cell purity representative of our manufacturing process has previously been reported to be over 90% [18]. The ovarian cancer cell line, Ovcar3, served as the TC line in order to measure the activity of the NK cells on a cancer cell type that is the focus of active clinical investigation for NK therapy [10]. In order to monitor NK cell killing of Ovcar3 cells, the molecule Calcein-AM was utilized. Calcein-AM exists as a non-fluorescent molecule prior to passively crossing cell membranes and being converted by intracellular esterases into a highly fluorescent compound that is retained by intact membranes resulting in fluorescently labeled Ovcar3 cells. Upon disruption of the cell membrane by NK cells, Calcein-AM is released from the cells. While this method has commonly been utilized in NK and T cell cytotoxicity assays with flow cytometry [29], here we utilized fluorescent microscopy to rapidly and reliably quantitate the Calcein-AM signal in the Ovcar3 cells.
Fluorescent microscopy-based cytotoxicity assay allows for measurement of direct tumor lysis by primary NK cells. a Schematic showing general workflow involved in the HTS assay b Schematic showing the detailed steps performed during the HTS process c Representative fluorescent microscopy images of Calcein-AM labeled Ovcar3 cells from the control groups utilized for the screen: Ovcar3 cells alone (left panel), neutral control (middle panel), and positive control (right panel) d) Representative images depicting the image processing and analysis used to determine target cell cytotoxicity
In order to develop the screen, we first optimized the incubation time needed for co-culture of NK and Ovcar3. We compared the standard 4 h co-culture of NK and TC with a 2 h co-culture and found that ~ 60% of killing occurred during the initial 2 h (Supplementary Fig. 1a). In order to increase the high-throughput capacity of the screening method, the 2 h time point was selected. To evaluate tolerable concentrations of DMSO, 15,000 NK cells per well were treated for 16 h with DMSO (0.25–1%) and assessed for viability using the Calcein-AM assay. NK cells were found to tolerate up to 0.5% DMSO treatment with no effect on cytotoxic activity or viability as compared to untreated NK cells (Supplementary Fig. 1b). Next, we established the following controls for the screen: 0.1% DMSO treated TC only (negative control), 0.1% DMSO treated NK cells co-cultured with TC at 1.5:1 effector to target (ET) ratio (neutral control), and 0.1% DMSO treated NK co-cultured with TC at a high ET ratio of 7.5:1 (positive control) (Fig. 1c).
We developed a method to quantitatively assess the NK cell cytotoxic effects on Calcein-AM labeled TC using imaging microscopy (Fig. 1d). In the first step of image processing, all objects are identified within a captured image using Cell Profiler software (v3.1.8). Next, cells are identified based on a size threshold to eliminate debris or large aggregates. Finally, live TCs are identified based on a live/dead fluorescent intensity threshold calculated per plate based on the neutral control (DMSO treated NK cells). Given these parameters, the fraction of live Ovcar3 target cells is calculated per well (fraction live = live TC number/total TC number). Prior to performing the full HTS, a pilot screen of two 384-well test plates from a Bioactive compound library was performed and resulted in Z’ scores of 0.759 and 0.805, which is indicative of an excellent assay[30].
Primary high-throughput screen
A high-throughput screen was performed using an 8000-small molecule diversity subset that is representative of the chemical space present in the UT Southwestern Chemical Library (200,000 compounds). To overcome NK cell heterogeneity among NK cell donors, a single batch of expanded primary human NK cells was used for the entire screen. NK cells were robustly expanded using irradiated NKF feeder cells to reach the appropriate numbers necessary for screening[18]. Prior to screening, expanded NK cells from this specific donor were tested to determine the optimal ET ratio of 1.5:1 to achieve a baseline percentage of target cell death at approximately 40% (Fig. 2a). To perform the screen, 15,000 viable NK cells were plated per well in 50 μL media containing 100 U/mL IL-2. Compounds were added to a final concentration of 5 μM per well (0.1% DMSO). DMSO was dispensed to all control wells (0.1% final concentration). After 16 h in 5% CO2, Ovcar3 cells (10,000 cells per well in 25 μl of media) were pre-labeled with Calcein-AM (stained with 1 μM Calcein-AM for 20 min at 37 °C) and subsequently added to all wells. The NK and Ovcar3 cells were co-cultured for 2 h at 37 °C in 5% CO2.
High throughput screening identified multiple small molecules that lead to enhanced NK cell cytotoxic activity. a NK cell dose curve to determine the optimal ET ratio for the screen (n = 3). b Histogram of the DMSO treated neutral control population from an exemplar plate from the pilot screen. After identification and intensity measurement, all cells in the 16 neutral control wells were binned by their total intensity measurement. The bimodal population represents a mixture of primarily bright live cells with a smaller fraction of darker dead cells. The saddle point in the histogram was chosen as the point in the intensity distribution that distinguished dark dead cells from bright live cells. c Quantile–quantile plot of pilot screen distribution of hits based on RZ score value
After the 2-h co-culture, the plates were imaged via fluorescent microscopy. All Ovcar3 cells were identified using a low global intensity threshold on the Calcein-AM channel. To classify live versus dead cells, the population intensity histogram for each neutral (DMSO) control well was determined. Since there is a population of dead cells in the neutral control wells from basal levels of NK cell lysis of Ovcar3 cells, the saddle point between the live (high Calcein-AM intensity) and dead (low Calcein-AM intensity) populations in each well is chosen as the filtering point to differentiate the two groups (Fig. 2b). A per-plate intensity cutoff value was calculated by averaging the intensity cutoff values for all the neutral control wells in each plate. This value was then used to classify all cells in each plate as dead or alive. The fraction of live Ovcar3 cells per well was then calculated. These values were then parsed into the Analyzer module of the Genedata Screener analysis software (v. 15, Genedata, Inc), where they were corrected for systematic errors such as row or column effects, and visually inspected for non-recurring plate effects [27].
A Z’ value was calculated for each plate. Subsequently, all wells were normalized to the robust median of the wells containing the chemical library (columns 3–22). An RZ score was then calculated for each compound [27] [31]. For the primary screen, a compound was deemed a hit if there was a > 10% increase in NK cell killing as compared to the robust median NK cell killing observed for all test wells on the relevant assay plate (Fig. 2c). Results from the primary screen are available on PubChem.
Validation of primary hits
A total of 30 compounds were identified as hits in the primary screen and were evaluated by follow-up studies. In confirmation testing (5 µM, 3 replicates per compound), 21 of the 30 compounds (70%) reproduced activities observed in the primary screen. In the same study, additional doses were tested (2.5, and 1 μM, 3 replicates per compound) and several of the compounds showed good dose-responsiveness (Fig. 3a). Next, we examined the possibility of false positive hits resulting from possible direct toxic effects of the compounds on Ovcar3 cells. As the NK cells were pretreated with the small molecules and the cells were diluted when the TC were added, the theoretical drug concentration in which the TC were exposed was reduced by 33% to 3.33, 1.65, and 0.66 μM and limited to 2 h of duration. To assess for direct toxic effects on TC, Ovcar3 cells were treated for 2 h and monitored with the Calcein-AM assay. There was no evidence of any direct toxicity of any of the validation screen drugs at concentrations ranging from 1 to 5 μM (Fig. 3b). To independently validate the results of the screening assay, a subset of compounds (Table1) was tested to assess effects on NK cell cytotoxicity using an independent and well-established luciferase-based cytotoxicity assay that monitors the loss of luminescence signal in TC (Fig. 3c). While all compounds increased NK cytotoxicity against Ovcar3 TC, compound 2 was selected for further biological studies. Additional hits validated in secondary screen can be found in Supplementary Table 1.
Compounds identified in HTS were confirmed via the primary HTS assay. a) Validation screen of selected compounds using the screening assay at the indicated concentrations (n = 3). Relative % activity is calculated by per well normalization to the number of cells in each well. The robust median well value across control wells is used to normalize the compound-containing well results and scale the data such that DMSO treated NK wells are at or near 0 while compound containing wells are relative to that control. b) Direct toxicity assessment of validated compounds on Ovcar3 target cells at the indicated concentrations (n = 3) in the primary HTS assay. Calcein-AM labeled Ovcar3 cells were treated with the compounds for 2 h and then assessed for cytotoxicity using the fluorescent microscopy (n = 3). c) Validation of selected hits using an orthogonal luciferase-based cytotoxicity assay that measures the loss of bioluminescence in luciferase transduced TC after co-culture with treated NK cells (n = 3)
Characterization of compound 2
To further characterize the activity of compound 2 (3-[(6-ethoxy-1,3-benzothiazol-2-yl) thio]-N-(4-methoxyphenyl) propenamide) in regulating NK cell activity, we performed time- and dose-dependent assays. Treatment of NK cells with compound 2 (C19 H20N2O3S2) resulted in a 77% increase in cell death at 24 h, with significant but smaller increases observed after 4 h and 10 h (Fig. 4a). Maximal increase in cell killing was observed after 24 h of treatment with 5 μM compound 2 against Ovcar3 TC compared to lower doses of 0.5 and 1 μM (Fig. 4b). Though NK cells are known to exhibit a high specificity in killing tumor cells as opposed to normal cells, it was not clear if this specificity is maintained after NK cell activation with compound 2. To assess for this property, cytotoxicity assays were performed using normal human T cells as TC, as opposed to cancer cells. In contrast to tumor cells, no enhancement of cytotoxic effects of compound 2 treated NK cells on normal human T cells were observed (Fig. 4c). Next, we assessed the effect of compound 2-treated NK cells on additional target cancer cells in addition to Ovcar3 cells. After a 24-h treatment of primary human NK cells with compound 2, we observed marked increases in NK cell cytotoxicity in an ET ratio-dependent fashion against RAJI (B cell lymphoma), HCT116 (Colon cancer) and HT29 (Colon cancer) cells (Fig. 4d). For example, NK cell cytotoxic activity increased by fivefold against RAJI and HCT116 cells at a 2:1 ET ratio and tenfold against HT29 cells after compound 2 treatment. Similar to Ovcar3 cells, both HCT116 and HT29 exhibited a dose dependent increase in TC death (Supplementary Fig. 3a). Finally, we characterized the phenotype of the NK cells after treatment with compound 2 by flow cytometry. Consistent with the increased cytotoxic activity of the compound 2-treated NK cells, the treated NK cells displayed a phenotype consistent with an activated state including induction of the cytotoxic mediators: perforin, granzyme, and the cytokines IFNγ, and TNFα (Fig. 4e) as compared to vehicle-treated cells.
Compound 2 enhances NK cell cytotoxicity against cancer cells, but not normal cells. a) Compound 2 enhances NK cell cytotoxicity in a time-dependent fashion. NK cells were treated with DMSO control or 5 μM Compound 2 and Ovcar3-luciferase cell killing using the luciferase-based cytotoxicity assay (n = 3). b) Compound 2 enhances NK cell cytotoxicity in a dose-dependent fashion. NK cells were treated with Compound 2 for 24 h and Ovcar3 cell killing was assessed using the luciferase-based cytotoxicity assay (n = 3). c) Compound 2 does not enhance NK cell cytotoxicity against primary human T cells. NK cells were treated with 5 μM Compound 2 for 24 h and assessed for cytotoxicity against T cells using the Calcein-AM microscopy-based cytotoxicity assay (n = 3). d) Compound 2 enhances NK cell-mediated cytotoxicity of the indicated cancer cell lines. NK cells were treated for 24 h with 5 μM compound 2 and assessed for cytotoxicity using the luciferase-based cytotoxicity assay (n = 3). e) NK cells exhibit phenotypic changes consistent with NK cell activation after treatment with compound 2. NK cells were treated with 5 μM of compound 2 or DMSO for 24 h and then profiled for the expression of the indicated markers by flow cytometry (n = 3). Compound-treated NK expression was normalized to the neutral control
Discussion
NK cells demonstrate high promise for adoptive cell therapy for cancer due to their ability to rapidly kill cancer cells with a high level of specificity for cancer versus normal cells. In addition, NK cells exhibit unique attributes as compared to more conventional T cell therapy, such as the lack of graft versus host disease with allogenic products and the ability of NK cells to target tumor cells without the necessity of specific antigen recognition [2]. NK cells in many cancer patients, such as AML patients, have impaired cytotoxic activity, further supporting the importance of NK cell activity in combatting cancers [13], 32. In contrast, highly active NK cells have been correlated with decreased tumor burden and better outcomes in cancer patients; NK activation is positively correlated with better outcomes in AML patients undergoing chemotherapy, as demonstrated by an activating ligand repertoire on AML blasts [7]. This further supports the importance of NK cell activation in favorable anti-tumor outcomes. Due to these observations, the discovery of novel pathways and small molecules that regulate NK cell activity is important.
Though adoptive NK cell therapy has demonstrated promise for cancer therapy, significant challenges remain in order to develop NK products that demonstrate sufficient clinical efficacy as most reported clinical trials describe limited and short lived benefits of NK products[33]–[36]. For example, a phase 2 clinical trial using adoptively infused NK cells for patients with AML and myelodysplastic syndrome demonstrated promise with two patients achieving a complete response (CR). The patients relapsed after 2 months, indicating the need to improve efficacy of NK adoptive therapy. A phase 1 clinical trial on 15 patients with non-small cell lung carcinoma (NSCLC) treated with IL-15 activated allogeneic NK cells reported two patients with partial response and six patients with disease stabilization with no observed side effects. These studies affirm the safety of adoptive NK therapy, while indicating a need to improve the activity of adoptive NK therapy.
In order to identify small molecules that enhance NK cell-mediated lysis of cancer cells, we developed and optimized a HTS assay. Screening strategies have more commonly been applied to identify new modulators of T cell receptor signaling and T cell activation [37]. Limited previous efforts have been reported to identify small molecule activators of NK cells, but these studies have relied primarily on low throughput assays and often utilized indirect markers (IFN-γ secretion) to measure NK cell cytotoxic activity [38]. These screens have also commonly utilized an NK cell line or primary NK cells from multiple donors. Previous efforts include screening 502 natural products using primary NK cells from three donors using cell lung cancer target cells [38]. This screen measured NK activation indirectly by assessing IFN-γ secretion by ELISA. Another screen tested 2,880 compounds from a natural product library using a luciferase based cell killing assay with lung cancer TC that assessed the impact of the compounds directly in the co-culture for a period of 24 h[26]. In another case, a genetic screen was performed on the NK92 cells line to identify modulators of NK92 cell line activity using RNAi [39]. Using a luminescence-based cytotoxicity assay, they identified specific genes in target cells that mediate antibody-dependent cellular cytotoxicity.
One obstacle to performing large scale screens in the past from primary cells was the challenge of procuring sufficient numbers of primary NK cells from the same donor. Due to the development of NK feeder cell lines that permit robust NK cell expansion, we have been able to implement a HTS using primary NK cells from a single donor to avoid issues with donor-to-donor variability. In addition, our screen utilizes an NK product that is highly clinically relevant as it is currently being utilized in clinical trials for relapsed/refractory cancer patients. We have further developed a direct readout for NK cell cytotoxicity using imaging that is rapid and reliable to support HTS efforts.
In order to validate our screening assay, we performed a screen of 8,000 small molecules. The screening assay was found to be highly robust with Z’ \(\ge \) 0.58 and led to the identification of 21 hits that increased NK cell killing by > 10% compared to the robust median NK cell killing observed for all test (chemical library) wells on the relevant assay plate. We further validated the reliability of the screen, by confirming the hits for the induction of NK cell cytotoxic activity through the performance of separate NK cell cytotoxic assays using different methodologies including a luminescence-based assay. Compound 2 was selected as our most promising small molecule because it reproducibly enhanced NK cytotoxicity. Finally, more detailed characterization of compound 2 was performed to further demonstrate its properties in inducing NK cell activation. Importantly, though the NK cell screen was performed using a single NK cell donor and Ovcar3 ovarian cancer cells, the secondary assays using compound 2 demonstrated that these results are not specific to a single NK donor or to ovarian cancer cells; NK cell hyperactivation was observed across a panel of blood cancer and solid tumor cell lines, as well as using multiple NK cell donors after their treatment with compound 2. Importantly, even though compound 2-treated NK cells demonstrate increased cytotoxicity against tumor cells, there was no increased cell lysis observed on primary T cells.
While the screening assay reported has high potential to identify novel compounds and pathways related to NK cell activation, the mechanisms of action of the compounds utilized for this screen are not known and further studies are needed to determine pathways involved in their biological activities. Further, additional biological studies including mouse tumor models are needed to further assess the promise of the NK cell activating compounds in vivo. It will be of particular interest for future developments to assess whether the compounds are also able to activate NK cells in vivo including both endogenous NK cells and adoptively infused NK cells. Overall, these results indicate that our HTS efforts can reliably identify compounds that stimulate NK cell hyperactivation against cancer cells. This screen has the potential to be used to not only contribute toward a greater understanding of NK cell biology, but also to assist in the development of therapeutic strategies to enhance NK cell activity for cancer patients.
Availability of data and material
The datasets generated during and/or analyzed during the current study are available on PubChem.
References
Stojanovic A, Correia MP, Cerwenka A (2013) Shaping of NK cell responses by the tumor microenvironment. Cancer Microenviron 6(2):135–146. https://doi.org/10.1007/s12307-012-0125-8
A. S. Chretien et al. 2017 NKp46 expression on NK cells as a prognostic and predictive biomarker for response to allo-SCT in patients with AML. Oncoimmunology. https://doi.org/10.1080/2162402X.2017.1307491.
Vitale M, Cantoni C, Pietra G, Mingari MC, Moretta L (2014) Effect of tumor cells and tumor microenvironment on NK-cell function. Eur J Immunol 44(6):1582–1592. https://doi.org/10.1002/eji.201344272
Dotiwala F et al (2016) Killer lymphocytes use granulysin, perforin and granzymes to kill intracellular parasites. Nat Med 22(2):210–216. https://doi.org/10.1038/nm.4023
Garg TK et al (2012) Highly activated and expanded natural killer cells for multiple myeloma immunotherapy. Haematologica 97(9):1348–1356. https://doi.org/10.3324/haematol.2011.056747
Liu E et al (2020) Use of CAR-transduced natural killer cells in CD19-positive lymphoid tumors. N Engl J Med 382(6):545–553. https://doi.org/10.1056/nejmoa1910607
Mastaglio S et al (2018) Natural killer receptor ligand expression on acute myeloid leukemia impacts survival and relapse after chemotherapy. Blood Adv 2(4):335–346. https://doi.org/10.1182/bloodadvances.2017015230
Plonquet A et al (2007) Peripheral blood natural killer cell count is associated with clinical outcome in patients with aaIPI 2–3 diffuse large B-cell lymphoma. Ann Oncol 18(7):1209–1215. https://doi.org/10.1093/annonc/mdm110
YXT Nadia Guerra, NT Joncker, FG, Augustine Choy, and DH Na Xiong, Susan Knoblaugh, Dragana Cado, Norman R Greenberg, and Raulet 2008 NKG2D-deficient mice are defective in tumor surveillance in models of spontaneous malignancy. Immunity 28(4): 571–580. https://doi.org/10.1016/j.immuni.2008.02.016.NKG2D-deficient.
Klapdor R et al (2017) Improved killing of ovarian cancer stem cells by combining a novel chimeric antigen receptor-based immunotherapy and chemotherapy. Hum Gene Ther 28(10):886–896. https://doi.org/10.1089/hum.2017.168
Garcia-Iglesias T et al (2009) Low NKp30, NKp46 and NKG2D expression and reduced cytotoxic activity on NK cells in cervical cancer and precursor lesions. BMC Cancer 9:1–8. https://doi.org/10.1186/1471-2407-9-186
L. Ruggeri et al.2002 Effectiveness of donor natural killer cell aloreactivity in mismatched hematopoietic transplants. Science 295(5562): 2097–2100. https://doi.org/10.1126/science.1068440.
Miller JS et al (2005) Successful adoptive transfer and in vivo expansion of human haploidentical NK cells in patients with cancer. Blood 105(8):3051–3057. https://doi.org/10.1182/blood-2004-07-2974
Siegel RL, Miller KD, Jemal A (2020) Cancer statistics, 2020. CA Cancer J Clin 70(1):7–30. https://doi.org/10.3322/caac.21590
Nham T et al (2018) Ex vivo-expanded NK cells from blood and ascites of ovarian cancer patients are cytotoxic against autologous primary ovarian cancer cells. Cancer Immunol Immunother 67(4):575–587. https://doi.org/10.1007/s00262-017-2112-x
Geller MA et al (2011) A phase II study of allogeneic natural killer cell therapy to treat patients with recurrent ovarian and breast cancer. Cytotherapy 13(1):98–107. https://doi.org/10.3109/14653249.2010.515582
C. J. Denman et al. 2012 Membrane-bound IL-21 promotes sustained Ex Vivo proliferation of human natural killer cells. PLoS One. https://doi.org/10.1371/journal.pone.0030264.
Ojo EO et al (2019) Membrane bound IL-21 based NK cell feeder cells drive robust expansion and metabolic activation of NK cells. Sci Rep 9(1):1–12. https://doi.org/10.1038/s41598-019-51287-6
Pinette A et al (2019) An IL-15-based super agonist ALT-803 enhances the NK cell response to cetuximab-treated squamous cell carcinoma of the head and neck. Cancer Immnuol Immunother 68(8):1379–1389. https://doi.org/10.1007/s00262-019-02372-2.An
Kerr WG, Chishol JD (2019) The next generation of immunotherapy for cancer: Small molecules could name big waves. J Immunol 202(1):11–19. https://doi.org/10.4049/jimmunol.1800991.the
h. sun and c. sun 2019 the rise of nk cell checkpoints as promising therapeutic targets in cancer immunotherapy. Front. Immunol 10: 1–14.
Otegbeye F et al (2018) Inhibiting TGF-beta signaling preserves the function of highly activated, in vitro expanded natural killer cells in AML and colon cancer models. PLoS ONE 13(1):1–13. https://doi.org/10.1371/journal.pone.0191358
Salih J et al (2010) The BCR/ABL-inhibitors Imatinib, nilotinib and dasatinib differentially affect NK cell reactivity. Int J Cancer 127(9):2119–2128. https://doi.org/10.1002/ijc.25233
Parameswaran R et al (2016) Repression of GSK3 restores NK cell cytotoxicity in AML patients. Nat Commun 7:1–11. https://doi.org/10.1038/ncomms11154
Pereira DA, Williams JA (2007) Origin and evolution of high throughput screening. Br J Pharmacol 152(1):53–61. https://doi.org/10.1038/sj.bjp.0707373
Xu Z et al (2020) A high-throughput assay for screening natural products that boost NK cell-mediated killing of cancer cells. Pharm Biol 58(1):357–366. https://doi.org/10.1080/13880209.2020.1748661
Zhijin Wu, Liu D, Sui Y (2008) Quantitative assessment of hit detection and confirmation in single and duplicate high-throughput screenings. J Biomol Screen 13(2):159–167. https://doi.org/10.1177/1087057107312628
Tang X et al (2018) Erratum: First-in-man clinical trial of CAR NK-92 cells: safety test of CD33-CAR NK-92 cells in patients with relapsed and refractory acute myeloid leukemia. Am J Cancer Res 8(9):1899
Jang YY et al (2012) An improved flow cytometry-based natural killer cytotoxicity assay involving calcein AM staining of effector cells. Ann Clin Lab Sci 42(1):42–49
An WF, Tolliday N (2010) Cell-based assays for high-throughput screening. Mol Biotechnol 45(2):180–186. https://doi.org/10.1007/s12033-010-9251-z
B. Chen et al., “Small molecule-mediated in Tissue Regeneration and Cancer,” vol. 5, no. 2, pp. 100–107, 2009. https://doi.org/10.1038/nchembio.137.Small.
Lichtenegger FS, Lorenz R, Gellhaus K, Hiddemann W, Beck B, Subklewe M (2014) Impaired NK cells and increased T regulatory cell numbers during cytotoxic maintenance therapy in AML. Leuk Res 38(8):964–969. https://doi.org/10.1016/j.leukres.2014.05.014
JP Veluchamy, N Kok, HJ van der Vliet, HMW Verheul, TD de Gruijl, and J Spanholtz 2017 The rise of allogeneic Natural killer cells as a platform for cancer immunotherapy: recent innovations and future developments. Front. Immunol. https://doi.org/10.3389/fimmu.2017.00631.
Shaffer BC et al (2016) Phase II study of haploidentical natural killer cell infusion for treatment of relapsed or persistent myeloid malignancies following allogeneic hematopoietic cell transplantation. Biol Blood Marrow Transplant 22(4):705–709. https://doi.org/10.1016/j.bbmt.2015.12.028
Iliopoulou EG et al (2010) A phase i trial of adoptive transfer of allogeneic natural killer cells in patients with advanced non-small cell lung cancer. Cancer Immunol Immunother 59(12):1781–1789. https://doi.org/10.1007/s00262-010-0904-3
Bachanova V et al (2010) Allogeneic natural killer cells for refractory lymphoma. Cancer Immunol Immunother 59(11):1739–1744. https://doi.org/10.1007/s00262-010-0896-z
Chen EW, Brzostek J, Gascoigne NRJ, Rybakin V (2018) Development of a screening strategy for new modulators of T cell receptor signaling and T cell activation. Sci Rep 8(1):2–10. https://doi.org/10.1038/s41598-018-28106-5
Gong C et al (2015) A high-throughput assay for screening of natural products that enhanced tumoricidal activity of NK cells. Biol Proced Online 17(1):6–13. https://doi.org/10.1186/s12575-015-0026-6
Murray J et al (2014) c-Abl modulates tumor cell sensitivity to antibody-dependent cellular cytotoxicity (ADCC). Cancer Immunol Res 2(12):1186–1198. https://doi.org/10.1158/2326-6066.CIR-14-0083.c-Abl
Acknowledgements
This research was supported by the following Case Comprehensive Cancer Center Shared Resources: Small Molecule Drug Development, Hematopoietic Biorepository and Cellular Therapy and Cytometry & Imaging Microscopy Shared Resource of the Case Comprehensive Cancer Center (P30CA043703). This work was also in part supported by an NIH-sponsored S10 grant (1S10OD018005-01 to B.A.P.) for the IN Cell Analyzer 6000 and R01CA259011. We thank Yong Han for performing LC/MS and HPLC analysis of compound 2.
Funding
P30CA043703, 1S10OD018005-01, R01CA259011. National Cancer Institute (US), P30CA043703,National Institutes of Health (US),1S10OD018005-01,Bruce Posner, National Cancer Institute,R01CA259011,David Wald
Author information
Authors and Affiliations
Contributions
G.L., K.M., H.W. and D.W. wrote and/or edited the manuscript. D.W., G.L., S.K., Y.F., and B.P. designed experiments. G.L., S.K., and H.N. performed the screening. D.W., Y.F., B.P., G.L., S.K., and H.C. analyzed the data. S.M. and Z.J. assisted with experiments.
Corresponding author
Ethics declarations
Conflicts of interest
The authors have no conflicts of interest to declare that are relevant to the content of this article.
Additional information
Publisher's Note
Springer Nature remains neutral with regard to jurisdictional claims in published maps and institutional affiliations.
Supplementary Information
Below is the link to the electronic supplementary material.
Rights and permissions
About this article
Cite this article
Lee, G., Karunanithi, S., Posner, B. et al. Chemical screening identifies novel small molecule activators of natural killer cell cytotoxicity against cancer cells. Cancer Immunol Immunother 71, 1671–1680 (2022). https://doi.org/10.1007/s00262-021-03117-w
Received:
Accepted:
Published:
Issue Date:
DOI: https://doi.org/10.1007/s00262-021-03117-w