Abstract
Aerial plant surface (phylloplane) is a primary key habitat for many microorganisms but is generally recognized as limited in nutrient resources. Pseudozyma antarctica, a nonpathogenic yeast, is commonly isolated from plant surfaces and characterized as an esterase producer with fatty acid assimilation ability. In order to elucidate the biological functions of these esterases, culture filtrate with high esterase activity (crude enzyme) of P. antarctica was applied onto leaves of tomato and Arabidopsis. These leaves showed a wilty phenotype, which is typically associated with water deficiency. Furthermore, we confirmed that crude enzyme-treated detached leaves clearly lost their water-holding ability. In treated leaves of both plants, genes associated to abscisic acid (ABA; a plant stress hormone responding osmotic stress) were activated and accumulation of ABA was confirmed in tomato plants. Microscopic observation of treated leaf surfaces revealed that cuticle layer covering the aerial epidermis of leaves became thinner. A gas chromatography-mass spectrometry (GC-MS) analysis exhibited that fatty acids with 16 and 18 carbon chains were released in larger amounts from treated leaf surfaces, indicating that the crude enzyme has ability to degrade lipid components of cuticle layer. Among the three esterases detected in the crude enzyme, lipase A, lipase B, and P. antarctica esterase (PaE), an in vitro enzyme assay using para-nitrophenyl palmitate as substrate demonstrated that PaE was the most responsible for the degradation. These results suggest that PaE has a potential role in the extraction of fatty acids from plant surfaces, making them available for the growth of phylloplane yeasts.
Similar content being viewed by others
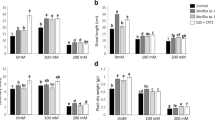
Explore related subjects
Discover the latest articles, news and stories from top researchers in related subjects.Avoid common mistakes on your manuscript.
Introduction
The outer side of epidermal cells of plants is covered with a waterproof waxy film called the cuticle. It consists of a cutin layer of lipophilic polyester matrix with long-chain fatty acids and wax that consists of mixtures of long-chain acyl lipids that are extremely hydrophobic (Chassot and Metraux 2005; Franke et al. 2005; Kolattukudy 1980; Yeats and Rose 2013). The cuticle layer serves as a defense barrier protecting plants against desiccation. Therefore, mutants that cannot synthesize the cuticle structure are reported to exhibit increased water evaporation (Buschhaus and Jetter 2012; Schonherr 1976).
The outer surface of the cuticle is one of the largest habitats for nonphytopathogenic microorganisms. Various bacteria were isolated at a ratio of 106 to 107 cells/cm2 (up to 108 cells/g) from leaves (Lindow and Brandl 2003). Basidiomycetous yeasts, such as genus Pseudozyma and Cryptococcus, were also isolated as colonists of phylloplane (Fonseca 2006; Kurtzman et al. 2011). Pseudozyma antarctica and various species of genus Cryptococcus (103 to 106 cells/g) were isolated from husks of rice cultivated in various areas in Japan (Kitamoto et al. 2011). In order to colonize plant surfaces, phylloplane microorganisms should obtain nutrients from plant surfaces. However, evidence for degradation of cuticle layer by nonpathogenic phylloplane microorganisms has not yet been found (Bashi and Fokkema 1977). P. antarctica has been previously named Candida antarctica and known to secrete strongly active lipase A (43 kDa) and lipase B (33 kDa) (Ishii 1988; Patkar et al. 1993) that are widely used in various industries (Nielsen et al. 1999) such as pitch control (Abo et al. 1992) and biodiesel production (Holm and Nielsen 2012). In addition, we isolated and identified a novel esterase, P. antarctica esterase (PaE), which exhibits efficient degrading ability for biodegradable polyester films, such as poly(ε-caprolactone), poly(butylene succinate), poly(butylene succinate-co-adipate), and poly(lactic acid) (Kitamoto et al. 2011; Shinozaki et al. 2013). Like the said biodegradable plastics, plant cutin is made of esterified organic acids that are solid at room temperature, although their components are not the same. In addition, the cuticle layer consists of various lipid structures. In the light of this background, we carried out this study to determine whether these yeasts have the ability to degrade cuticle layer.
Recently, we succeeded in preparing concentrated PaE in culture broth of P. antarctica by using xylose as carbon source (Watanabe et al. 2014). In this study, we first demonstrated that treatment of surfaces of potted plant leaves and detached leaves with the culture filtrate of P. antarctica (crude enzyme solution) resulted to water loss in these plants. Prompted by this observation, we examined the plant stress responses and morphological changes of leaf surfaces following treatment with the crude enzyme. Then, we chemically analyzed the compositions of total fatty acids that were released from leaf surfaces in the crude enzyme and control treatments. After showing that fatty acids were released from leaf surfaces by crude enzyme treatment, fatty acid ester degradation activity of each esterase in the crude enzyme was analyzed to determine which esterase contributes to the leaf surface degradation.
Material and methods
Plant material and growth condition
In this study, Arabidopsis thaliana (ecotype Col-0) and Solanum lycopersicum (L.) cv. ‘MicroTom’ of the family Brassicaceae and Solanaceae, respectively, were used as test plants. Tomato plants were grown on a mixture of soil which consisted of culture soil (Sakata super mix A, Sakata Seed, Yokohama, Japan) and vermiculite (1:1) under white fluorescent light (2.4 klux) in a 16-h light/8-h dark photoperiod at 25 °C with 40–50 % relative humidity (RH) for 4 weeks. A. thaliana (ecotype Col-0) plants were grown on vermiculite covered with perlite under white fluorescent light (1.8 klux) in a 12-h light/12-h dark photoperiod at 22 °C with 70–80 % humidity (RH) for 3 weeks.
Preparation of crude enzyme solution of P. antarctica
The crude enzyme solution used in this study was prepared by filtering 72-h xylose-fed batch culture of P. antarctica GB-4(0)-HPM7 cultivated in a jar fermentor. The culture condition for overproduction of PaE was followed as described previously (Watanabe et al. 2014). This strain, GB-4(0)-HPM7, derived from strain GB-4(0) (Kitamoto et al. 2011), was selected as a less-foam-forming mutant in our laboratory and was deposited in the NIAS Genebank at the National Institute of Agrobiological Sciences, Japan (accession number, MAFF 307000). The predicted amino acid sequence of PaE of this strain was confirmed as having 100 % similarity with that of type strain P. antarctica JCM10317 (DM067526) (Shinozaki et al. 2013).
Evaluation of PaE activity in crude enzyme solution
PaE activity of crude enzyme solution was evaluated by considering the decrement of turbidity of emulsified poly(butylene succinate-co-adipate) (PBSA) solution (Bionolle EM-301, average molecular weight 1.2 to 1.5 × 105, Showa Denko K. K) as described previously (Kitamoto et al. 2011). Among the three esterases in the crude enzyme solution, PaE was reported to be the one responsible for the degradation activity for PBSA (Kitamoto et al. 2011). One unit of PBSA degradation activity per 1 ml of crude enzyme solution was defined as a 1-U decrease in absorbance at 660 nm min−1 at 30 °C, pH 6.8.
Treatment of potted plant and detached leaves with crude enzyme solution
PaE exhibits a high relative esterase activity under alkaline condition (Shinozaki et al. 2013). So, in this study, crude enzyme solution containing 8-U PaE activity was generally suspended in 20 mM Tris-HCl buffer (pH 8.8) just before use. Negative control solution was prepared by autoclaving the same crude enzyme solution at 121 °C, 15 min, and used after confirming that the PaE was inactivated.
Crude enzyme solution was applied on MicroTom and Arabidopsis plants as follows: About 600 μl of crude enzyme solution was sprayed over the upper side of each leaf by using a pump spray, with a total of 10 ml on each MicroTom plant and 5 ml on each Arabidopsis plant. Three of each potted plant species with the same treatment were incubated in a cultivated field at an average temperature of 28.6 °C for 7 days without controlled water supply (natural condition). The experiment was done from July 27 to August 2, 2013(after rainy season), in Tsukuba, Japan (http://www.data.jma.go.jp/).
Detached third or fourth leaves of MicroTom and rosette leaves of Arabidopsis plants were used in crude enzyme treatment, which was done in two ways described as follows:
-
Spray treatment. About 600 μl of crude enzyme solution was sprayed over the upper side of each cut leaf by using a pump spray. Then, the leaves were kept under high humidity in closed container for 3 or 7 days at their respective growth temperature.
-
Submergence treatment. Each bundle of four MicroTom leaves or eight Arabidopsis rosette leaves (ca. 1 g) was carefully submerged in 10 ml of crude enzyme solution or control solution placed in a 20-ml glass vial, avoiding the immersion of the cut surface of petiole in the crude enzyme solution. The vials were incubated at 25 °C with shaking at 165 rpm for 16 or 24 h.
Effect of crude enzyme solution on water-holding ability of leaves
Water-holding ability of leaves was confirmed based on weight loss in leaves due to water evaporation by using general methods (Bessire et al. 2007; Buschhaus and Jetter 2012; Cominelli et al. 2008) after submergence treatment in crude enzyme or inactivated enzyme solution for 24 h as follows: Each leaf was briefly sandwiched between soft wipers (Kim Towel, Nippon Paper Crecia Co., Ltd, Tokyo, Japan) to remove leaf surface moisture and then separately placed in a plate and weighed (initial weight). These plates were kept in a laboratory bench at 25 °C under about 28 % RH and were weighed at different time points (0, 15, 30, 45, 60, and 90 min after treatment). Water loss was calculated based on the rate of weight decrease. Four individual samples were used in each trial.
RNA isolation and quantitative qRT-PCR analysis
Leaf samples spray treated with active crude enzyme or inactivated enzyme solution were incubated for 3 days as described above. Thereafter, their weights were measured after removing the surface moisture and then were frozen by liquid nitrogen. Six frozen leaf samples were separately ground using a mortar and pestle. Total RNA of each sample was extracted from the resulting powder with TRIzol® reagent (Invitrogen, San Diego, CA) as recommended by the supplier. qRT-PCR was performed on 1 μg of total RNA using iScript™ cDNA Synthesis Kit (Bio-Rad, Hercules, CA, USA) and iQ™ SYBR® Green supermix (Bio-Rad, Hercules, CA, USA) as recommended by the supplier. To study the expression of drought-responsive genes, specific primers were designed as shown in Table 1: SlNCED1 (AJ439079), SlCYP707A2 (HQ008774), and SlAIM1 (NM_001247196) for MicroTom and AtNCED3 (NM_112304) and AtCYP707A3 (NM_180805) for Arabidopsis. Control reactions to normalize RT-PCR amplification were run using the MicroTom Actin (SlACT) gene (FJ532351) and Arabidopsis AtCBP20 gene (AF140219). AtCBP20 gene encodes a subunit of the cap-binding protein engaged in RNA metabolism and is constitutively expressed in all tissues of Arabidopsis (Kmieciak et al. 2002).
PCR was carried out on a Real-Time PCR System CFX96 (Bio-Rad, Hercules, CA, USA). The thermal cycling program was as follows: 95 °C for 60 s; 45 cycles of 95 °C for 15 s and 60 °C for 30 s.
Quantification of ABA
Leaf samples were prepared as described for RNA quantification. Extraction and quantification of abscisic acid (ABA) in frozen leaf samples were performed essentially as described previously (Baldwin et al. 1997; Seo et al. 1995). The amount of ABA in each of the six pieces of leaves was separately determined.
Observation of plant surface by scanning electron microscopy (SEM)
Leaf samples spray treated with crude enzyme were incubated for 7 days as described above. Samples from four independent trials for each treatment were cut into 1 cm × 1 cm square sections and then were fixed with 2 % glutaraldehyde in 0.1 M phosphate buffer (pH 7.4) overnight at room temperature. The fixed sample was dehydrated with a series of increasing ethanol concentration (30 to 100 %). Thereafter, samples were critical point-dried with CO2 gas using HCP-2 (HITACHI, Tokyo, Japan) and were mounted on aluminum stage and sputter coated with platinum particles by E-1010 (HITACHI, Tokyo, Japan). Samples were observed under the JMS-5610LV microscope (JEOL, Tokyo, Japan) with an accelerating voltage of 15 kV, and the resulting images were captured digitally.
Light microscopic observation of esterase-treated leaf sections
After leaf submergence in crude enzyme solution for 24 h, each leaf was cut to about 1 cm × 1 cm square sections. Samples were held between slit of pith and sectioned by razor manually. The cuticle sections were stained with Sudan III (Sigma-Aldrich) according to Buda et al. (2009) with some modifications as follows: Leaf sections were soaked in the filtered Sudan III solution (2 % w/v in 70 % ethanol) for 8 min and were then rinsed first with 50 % ethanol, followed by distilled water twice. Stained sections were mounted and observed under an ECLIPSE 80i light microscope with DS-Fi1 CCD camera with DS-L2 capture system (Nikon, Tokyo, Japan).
Extraction and analysis of total free fatty acids released from crude enzyme-treated leaves
Leaves of MicroTom or Arabidopsis (ca. 1 g) were submerged in 10 ml of crude enzyme reaction solution for 16 h (four replicates for each treatment). After removing the leaves from the reaction bottle, free fatty acids (FFAs) were extracted from crude enzyme solution with 5 ml of n-hexane, and the n-hexane fraction was evaporated under N2 flow and resuspended in 50 μl of methanol. Total FFAs contents in the resuspended solution were measured by enzymatic reaction with acyl-CoA oxidase of Pseudomonas sp. using nonesterified fatty acid (NEFA) C Kit (Wako, Osaka, Japan) according to the manufacturer’s protocol. Furthermore, the FFAs released from enzyme-treated leaf surfaces were analyzed and characterized by gas chromatography-mass spectrometry (GC-MS) as follows: An aliquot of the reaction solution (2 ml), where the leaves were submerged for 16 h as described above, was diluted with H2O (2 ml) and extracted with tert-butyl methyl ether (3 × 2 ml). The organic phase was dried over sodium sulfate and concentrated. FFAs were purified by silica gel column chromatography (Wako Gel C-200, Wako; 0.2 g) using 2 ml of 2 % acetic acid in diethyl ether as an eluant and then converted to methyl esters (Ichihara and Fukubayashi 2009) prior to GC-MS analyses. GC-MS conditions were described in our previous paper (Tabata et al. 2012).
Separation of esterases from crude enzyme solution by column chromatography
Crude enzyme solution (60 ml) was concentrated to approximately 2.5 ml (ca. 24-fold concentration) simultaneously with an exchange of the solvent to 20 mM Tris-HCl buffer (pH 8.0) using Amicon Ultra-15 Centrifugal Filter Unit with Ultracel-3K membrane (Millipore, Billerica, MA). The concentrated crude enzyme solution was filtered using a 0.2-μm pore-sized membrane (Ultrafree-MC, Millipore). A part of the filtrate (1 ml) was applied to a Mono Q HR 5/5 column (GE Healthcare Life Science, Little Chalfont, UK). Proteins were eluted by NaCl concentration gradient in 20 mM Tris-HCl buffer (pH 8.0) at a flow rate of 0.5 ml/min with a linear gradient of NaCl (0–1 M). The solvent of the flow-through fraction (10 ml) was exchanged to 50 mM phosphate buffer (pH 7.13) containing 1.2 M ammonium sulfate and concentrated to approximately 0.7 ml as described above. A part of the concentrate (0.35 ml) was applied to a Phenyl Superose HR 5/5 column (GE Healthcare Life Science), and the proteins were eluted by 50 mM phosphate buffer (pH 7.13) with a linear gradient of ammonium sulfate (1.2–0 M) at a flow rate of 0.5 ml/min. After washing the column with 5 ml of H2O, tightly adsorbed proteins were eluted with 500 μl of 40 % (v/v) acetic acid. The solvent of the 40 % acetic acid eluate was immediately exchanged to H2O and concentrated to 260 μl using Amicon Ultra-0.5 Centrifugal Filter Unit with Ultracel-3K membrane (Millipore).
Determination of esterase activity of lipases and PaE in column-separated fractions
Esterase activity of fractionated culture filtrate was monitored with α-naphthyl butyrate (Sigma-Aldrich) as substrate in 25 mM HEPES-NaOH buffer pH 7.20; the released naphthol reacted with the Fast Blue B Salt (MP Biomedicals, LLC Illkirch, France) producing purple color and was detected at 610 nm. The purity of enzyme was checked after separation of column fractionate by sodium dodecyl sulfate-polyacrylamide gel electrophoresis (SDS-PAGE) using 14.1 % separating gel, as described by Laemmli (Laemmli 1970), and silver staining (Daiichi Kagaku Yakuhin, Tokyo, Japan). SDS-PAGE molecular weight standards (Broad Range, Bio-Rad, Hercules, CA) were used. Zymograms of esterase were also visualized in SDS-PAGE gel as described by Karpushova et al. (Karpushova et al. 2005), except that α-naphthyl butyrate was used as substrate. Recombinant lipases A and B of P. antarctica produced by Aspergillus oryzae (L-3420 and L3170, respectively, (Sigma-Aldrich)) were used as control after their total protein concentrations were determined by Quick Start Bradford protein assay with bovine gamma globulin standard (Bio-Rad). Wide-view prestained protein size marker (Wako Pure Chemical Industries, Ltd, Osaka, Japan) was used for molecular weight determination.
In the fraction where α-naphthyl butyrate degradation activity was detected, the degradation activity of cast film of para-nitrophenylpalmitate (pNP-C16) (Sigma-Aldrich) was evaluated as follows: pNP-C16 was dissolved in 3.3 mM chloroform, and the mixture was applied on a water-repellent printing glass slide (Matsunami Glass Ind., Ltd, Osaka, Japan) at 20 μl in each well (6-mm diameter) and air-dried at room temperature. Each column fraction (5 μl) was dissolved with 45 μl of 55 mM Tris-HCl, pH 8.8, and placed onto the pNP-C16 films. The glass slide was put inside a small humid container and incubated at 30 °C for 1 h. The reaction solution (40 μl) was collected into a 96-well microplate, and the OD400 values were measured using a multi-spectrometer (Dainippon, Osaka, Japan). The OD400 values of the reaction solutions were obtained after subtracting the value for the control (buffer solution without enzymes). The analyses of samples were performed in triplicate.
Results
Effect of crude enzyme treatment on phenotypes of potted plants and detached leaves
At the initiation of this study, potted plants of MicroTom and Arabidopsis were spray treated with crude enzyme solution, and then, after cultivation under natural drought conditions for 7 days, the phenotypes were compared with nontreated plants. Photographs of treated plants are presented in Fig. 1. The color of the MicroTom leaves at the bottom of stem turned yellow, indicating water deficiency during cultivation. More severe defect was observed in crude enzyme-treated potted plants. They were withered and lost most of their leaves. On the other hand, only a slight defect was observed in Arabidopsis potted plants treated with crude enzyme, but they were more wilted compared to the control plants.
Phenotypes of crude enzyme-treated potted plants. MicroTom plant treated with inactivated enzyme (control sample) (a) and active crude enzyme (b). Arabidopsis plant (control sample) (c) and crude enzyme-treated sample (d). After crude enzyme treatment, potted plants were grown for 7 days under natural conditions
Detached leaves of MicroTom and Arabidopsis were spray treated with crude enzyme for more detailed study. Similar to the results in potted plants, crude enzyme-treated leaves became wilted faster than in control samples. The progression of chlorophyll loss was accompanied by increased browning of leaves. Photographs of typical phenotypes of treated leaves are presented in Fig. 2a, b, and for comparison, plants treated with inactivated crude enzymes are also shown.
Effect of crude enzyme treatment on water-holding ability of leaves
In the light of the above results, we considered that crude enzyme treatment could have impaired the plant surface function as water diffusion barrier, leading to severe water deficiency in plants. To evaluate the water evaporation rate in enzyme-treated leaves, the weight loss of MicroTom and Arabidopsis leaves was monitored after the submergence treatment (Fig. 3a, b). The ratio of weight loss in the enzyme-treated leaves was found higher than that in the control samples. Significant difference in weight loss was observed between the control and the treated MicroTom leaves incubated for over 60 min. Similar effect was observed in Arabidopsis leaves incubated for over 30 min. These results indicated that leaves treated with crude enzyme had increased water loss, in other words, decreased water-holding ability under drought condition.
Water-holding ability of leaves treated with crude enzyme. Time course of leaf weight reduction ratio of control (open circles) and crude enzyme-treated leaves (closed circles) of MicroTom (a) and Arabidopsis (b). Asterisks indicate significant differences between enzyme-treated samples compared with their corresponding control (Student’s t test; *P < 0.05, **P < 0.01). Relative leaf weight was expressed as percentage based on the initial fresh weight. The average of four biological replicates was presented at each time point. Bars indicate SE of the mean
Evaluation of change in drought stress signaling and ABA content of leaves
To confirm the drought stress response of crude enzyme-treated leaves, we measured the expression levels of selected inducible genes for drought stress signaling using quantitative real-time PCR (Fig. 4a, b). 9-cis-Epoxycarotenoid dioxygenase (NCED) is a gene for the enzyme involved in the biosynthesis of phytohormone ABA, CYP707 (ABA 8′-hydroxylase) is for an ABA-degrading enzyme (Iuchi et al. 2001; Nitsch et al. 2009; Saito et al. 2004), and ABA-induced Myb (AIM) is for ABA signaling transcription factor (Abuqamar et al. 2009). These genes are known to show increased expression levels upon exposure to drought stress. Our results with MicroTom showed that all of the genes (SlNCED, SlCYP707A1, and SlAIM) were expressed more than twice as much as that of control samples (Fig. 4a), with SlNCED1 and SlCYP707A1 having particularly significantly higher expression levels. In Arabidopsis leaves, the expression levels of the marker genes (AtNCED3 and AtCYP707A3) were also elevated to more than twice, but significant increment was observed in the AtNCED3 gene (Fig. 4b).
ABA signaling by PaE treatment. Relative transcript levels analyzed by qRT-PCR of SlNCED1, SlCYP707A1, and SlAIM in PaE-treated MicroTom leaves and control treatment (a) and AtNCED3 and AtCYP707A3 in PaE-treated Arabidopsis rosettes and control treatment (b). SlACT and AtCBP20 were used as the internal control. Data represent mean ± SE of three replicates. Measurement of ABA in leaves of MicroTom (c) and rosettes of Arabidopsis (d) present in each treatment solution. Each value represents the mean ± SE of six replicates. Asterisks indicate significant differences between enzyme-treated samples compared with their corresponding controls (Student’s t test; *P < 0.05, **P < 0.01)
As the NCED was expressed in both plants, the amount of accumulated ABA was also measured. The results obtained showed that enzyme-treated MicroTom leaves accumulated significant amount of ABA (Fig. 4c). On the other hand, no difference in accumulation of ABA was observed in treated Arabidopsis leaves compared to the control (Fig. 4d).
Microscopic observation of surface and cross section of crude enzyme-treated leaves
Micromorphological properties of leaf surfaces spray treated with crude enzyme or inactivated crude enzyme were observed under SEM. Typical images of MicroTom and Arabidopsis leaf surfaces are presented in Fig. 5. In the MicroTom samples (Fig. 5a, b), the surfaces of control leaves were covered with wrinkled film presumed to be the cuticle layer, and the grooves formed by the junctions of epidermal cells were shallow (Fig. 5a). In contrast, the surfaces of enzyme-treated samples were smooth with less wrinkled film and the grooves between the epidermal cells were much deeper than those of the control samples (Fig. 5b, arrowhead). Similarly, the surfaces of the enzyme-treated Arabidopsis leaves also formed deeper grooves between epidermal cells (Fig. 5d, arrowhead) compared to those of the control samples (Fig. 5c). Wrinkling of the leaf surface was more prominent in the enzyme-treated samples.
Scanning electron micrograph of plant leaf surfaces treated with crude enzyme solution. Typical image of crude enzyme-treated leaves of MicroTom (b) and Arabidopsis (d). Control samples (a, c) were treated with inactivated crude enzyme solution. Arrowheads indicate grooves observed between packed cells. Scale bars = 10 μm
To clarify the effect of treatment of crude enzyme on the architecture of cuticle, a cross-sectional view of leaf surfaces of MicroTom and Arabidopsis was observed after staining the cuticle layer with Sudan III. Typical images are presented in Fig. 6. Sudan III, which stains lipophilic structure, did not generate background staining by occupying aqueous environments. Under the light microscope, red-stained thin layer, believed to be cuticle layer, was observed at the outer side of epidermal cells in the samples treated with inactivated enzyme (Fig. 6c, g, open triangles). In contrast, the crude enzyme-treated samples showed fuzzy red-stained layers (Fig. 6d, h, closed triangles), which were much thinner and sometimes were not clearly visible.
Cross-sectional image of crude enzyme-treated leaves. Histological observation of cross sections of Sudan III-stained cuticle layer of enzyme-treated MicroTom and Arabidopsis leaves. Cross sections of control samples and their magnified images: MicroTom control (a, c) and Arabidopsis (e, g). Crude enzyme-treated section and their magnified images: MicroTom (b, d) and Arabidopsis (f, h). The cuticle layer was stained with red (open triangles). Similar observations were made in sections of three independent trials. The experiment was repeated with similar results. Scale bars = 10 μm
Chemical analysis of lipophilic compounds released by crude enzyme treatment
Given the fact that fatty acids are the major components of cuticle layer of tomato (Nadakuduti et al. 2012) and Arabidopsis (Franke et al. 2005) leaves, we considered that esterases of P. antarctica may have the ability to release free fatty acids (FFAs) from plant surfaces. We, thus, quantified the total amount of FFAs released by crude enzyme treatment as described previously. Compared with the leaf sample treated with inactivated crude enzyme, a significant increase in FFAs contents were observed in the reaction solution of MicroTom leaves treated with active crude enzyme (crude enzyme 16.9 mEq/L and control 5.0 mEq/L). A little increment of FFAs contents were also found in the reaction solution of Arabidopsis leaves, although the amounts were not statistically significant (crude enzyme 6.3 mEq/L and control 5.2 mEq/L).
GC-MS analyses of MicroTom FFA components revealed that three to four times more hexadecanoic (palmitic, C16) or octadecanoic (stearic, C18) acids were released in the active enzyme solutions (C16: F 1,6 = 14.1, P = 0.0095; C18: F 1,6 = 18.8, P = 0.0049; Fig. 7a) than in control solution. Moreover, unsaturated FFAs (hexadecatrienoic acid, C16:3, and octadecatrienoic acid, C18:3) were characteristically detected in active enzyme solutions (Fig. 7a). A consistent result was observed in FFAs from Arabidopsis leaves; significantly larger amounts of saturated and unsaturated C16/C18 acids were detected in active enzyme solutions (Fig. 7b).
Chemical analysis of free fatty acids released by crude enzyme treatment. Free fatty acids (FFAs) released from plant leaf surface wax upon treatment with enzyme solutions (crude enzyme and control: inactivated enzyme). Characterization of FFAs from MicroTom (a) and Arabidopsis (b) by GC-MS analyses. Asterisks indicate significant large amounts of FFAs in PaE solutions (ANOVA; *P < 0.05). C8, C9, C12, C14, C15, C16, C16:3, C17, C18, C18:1, C18:3, and C20 indicate octanoic, nonanoic, dodecanoic, tetradecanoic, pentadecanoic, hexadecanoic, hexadecatrienoic, heptadecanoic, octadecanoic, octadecenoic, octadecatrienoic, and eicosanoic acid, respectively
Determination of esterase activity of lipases and PaE in column-separated fraction
Esterases in crude enzyme solution were separated by column chromatography and evaluated by measuring α-naphthyl-butyrate degradation activity. Based on the activity in the separated fractions, 84 % of esterases were recovered from the crude enzyme solution. Each eluted fraction with maximum α-naphthyl-butyrate degradation activity, i.e., fraction 5 (total of 25 fractions) from Mono Q column, fraction 20 (total of 20 fractions) from Phenyl Superose column, and fraction eluted with 40 % acetic acid from Phenyl Superose column, was separated by SDS-PAGE (Fig. 8). The results of silver staining of proteins (Fig. 8a) and zymography of degradation activity for α-naphthyl-butyrate (Fig. 8b) showed that the esterase activities in bound/elution fraction from Mono Q column (lane 6) and Phenyl Superose column (lane 7) had electrophoretic mobilities identical to lipase A (lane 4) and lipase B (lane 5). PaE was tightly adsorbed to Phenyl Superose HR 5/5 column but was successfully eluted with 40 % acetic acid as the major protein in this eluate (lane 3). In vitro assay for pNP-C16 degradation activity of separated fractions revealed that almost all of the relative pNP-C16 degradation activity (99.7 %) was detected in the fraction eluted with 40 % acetic acid from Phenyl Superose column.
SDS-PAGE and zymography of esterases of P. antarctica. SDS-PAGE (a) and zymography (b) of the purified esterases from P. antarctica. Lane M, molecular weight protein markers; lane 1, concentrated culture filtrate (0.2 μl); lane 2, flow-through fraction from Mono Q column (0.2 μl); lane 3, elution with acetic acid from Phenyl Superose column (0.2 μl); lane 4, standard lipase A (0.13 μg (a) and 1.3 μg (b)); lane 5, standard lipase B (0.07 μg (a) and 0.7 μg (b)); lane 6, eluted fraction 5 from Mono Q column (5 μl); lane 7, eluted fraction 20 from Phenyl Superose column (5 μl). In each result presented in a and b, all the samples were loaded in the same gel and indicated by silver staining (a) and by α-naphthyl-butyrate and Fast Blue B (b). In the photograph of gel (b) lanes 6 and 7, the intensity levels of the image shadows, midtones, and highlights were adjusted from 0, 1.00, 255 to 100, 0.30, 255, respectively, because the original images were not clearly visible. Black, white, and gray arrow heads indicated the position of lipase A, lipase B, and PaE, respectively
Discussion
Our results presented here demonstrated that PaE of P. antarctica had physiological and morphological effects on plant surfaces and released saturated and unsaturated FFAs.
At the beginning of this study, we applied the culture filtrate (crude enzyme solution) on plant surfaces and found that potted plants spray treated with culture filtrate of P. antarctica (crude enzyme) became easily wilted under drought condition (Fig. 1). Detached leaves with the same treatment also became wilted (Fig. 2). This phenotype indicated that crude enzyme treatment impaired the water transpiration barrier function of plant surfaces leading to weight loss (Fig. 3).
We considered that plants themselves could also detect their own dehydrated state. In this regard, we looked into the possible involvement of ABA accumulation and induction of the ABA-dependent stress signaling pathway in the detached leaves treated with crude enzyme. It has been widely reported that drought induces accumulation of ABA in plants as a stress response (Finkelstein 2013). We also observed similar phenomenon in the leaves of MicroTom where ABA accumulation was significantly enhanced by the crude enzyme treatment (Fig. 4c). On the other hand, in Arabidopsis, no significant difference in the amount of ABA produced was detected (Fig. 4d). L'Haridon et al. (2011) reported that wounded Arabidopsis leaves did not accumulate ABA under humid condition, as was adopted in this study. Even so, phenotypic symptoms in response to osmotic stress were observed in Arabidopsis leaves, as well as MicroTom leaves (Figs. 1 and 3). Furthermore, transcription levels of genes involved in ABA biosynthesis (SlNCED and AtNCED) were found to be significantly higher in both treated MicroTom and Arabidopsis leaves than in the control treatments, respectively (Fig. 4a, b). Additionally, the expression level of ABA hydroxylase gene (CYP707A), whose product functions at the final step of ABA signaling, was also enhanced in both plants (Fig. 4a, b).
The phenotypes, i.e., fast drying of leaves and accumulation of ABA, were also reported in several Arabidopsis and Oryza sativa mutants with defective leaf surface structures (Bessire et al. 2007; Park et al. 2010). In this study, the crude enzyme treatment of the test plants also caused change in their leaf surface morphology and the formation of deep grooves between the epidermal cells, as observed under SEM (Fig. 5, arrows). Since this crude enzyme has a strong esterase activity, we supposed that it could have possibly acted on the esterified lipid structure of plant surface (cuticle) to effect the said changes. It was reported that cross-sectional images revealed defect in the cuticle architecture of cutin-deficient mutants of tomato fruit (Buda et al. 2009; Isaacson et al. 2009), leaves of Arabidopsis (Bessire et al. 2007), and leaves of Oryza sativa (Park et al. 2010). A similar phenotype caused by expression of fungal cutinase gene of phytopathogens was also reported in transgenic Arabidopsis (Sieber et al. 2000). Optical microscopy of Sudan III-stained leaves revealed the obvious thinning of cuticle layer in enzyme-treated leaf (Fig. 6, closed triangles), which was not observed in control leaf. These results suggest that treatment with crude enzyme from P. antarctica had thinned the cuticle layer and decreased the retention ability of water in leaves.
Accordingly, we further tried to verify a hypothesis which claims that esterases from P. antarctica degrade ester bonds of leaf cuticle components to release FFAs. With the crude enzyme treatment, we detected an increase in the total amount of released FFAs, which were eluted in the reaction solution. The major FFAs released in significantly larger amounts in both Arabidopsis and tomato were saturated and unsaturated C16 and C18 acids (Fig. 7a, b). FFAs were generally detected in hydrolyzed cuticle layer of various plant surfaces (Riederer and Schonherr 1986). Nadakuduti et al. (2012) also reported the presence of FFAs in both cutin layer and wax layer of tomato leaves (Nadakuduti et al. 2012). We also showed that 99.7 % of the degradation activity against pNP-C16 was due to PaE present in the crude enzyme solution at pH 8.8 (Fig. 8). These results indicated that C16 and C18 acids were released from cuticle layer of leaves of both tomato and Arabidopsis upon treatment with crude enzyme of P. antarctica and that the major activity was attributed to PaE.
While PaE is also considered as a cutinase-like enzyme based on its weak structural similarity with filamentous fungal cutinase (Chen et al. 2013; Shinozaki et al. 2013), the major components of cutin, such as hydroxyl fatty acids of tomato (Nadakuduti et al. 2012) and dicarboxylic fatty acids of Arabidopsis (Franke et al. 2005), were not detected in this study, even though we tried various extraction/detection methods targeting oxygenized fatty acids. This result coincided with the characteristic of nonpathogenic phyllosphere yeasts that do not penetrate into the host (Bashi and Fokkema 1977).
Phylloplane has been considered to be a nutrient-poor habitat for many microorganisms because of the difficulty of leaf surfaces to exude nutrients due to the barrier posed by the cuticle layer (Fonseca 2006; Lindow and Brandl 2003). Strains of Pseudozyma are often isolated from leaf surfaces (Kurtzman et al. 2011) and were reported to have ability to assimilate natural triglycerides as well as to secrete three esterases, namely, lipases A and B and PaE (Ishii 1988; Kitamoto et al. 2011). As described above, the esterase (mainly PaE) of the P. antarctica strain used in this study exhibited strong activity which released FFAs from the cuticle layer of tomato and Arabidopsis leaves under laboratory conditions. This result, which is congruent with those of the abovementioned reports, suggests that if strains of Pseudozyma could secrete esterases on leaf surfaces, they will be able to obtain FFAs as cleavage products from the cuticle layer components of leaves. Accordingly, phylloplane could become an abundant carbon resource and, thus, can be considered as a good environment for the growth of esterase-producing yeasts.
References
Abo M, Fujita Y, Itami R, Matsukura M (1992) Method for avoiding pitch troubles by use of thermostable lipase. WO1992013130
Abuqamar S, Luo H, Laluk K, Mickelbart MV, Mengiste T (2009) Crosstalk between biotic and abiotic stress responses in tomato is mediated by the AIM1 transcription factor. Plant J 58(2):347–360. doi:10.1111/j.1365-313X.2008.03783.x
Baldwin IT, Zhang Z-P, Diab N, Ohnmeiss TE, McCloud ES, Lynds GY, Schmelz EA (1997) Quantification, correlations and manipulations of wound-induced changes in jasmonic acid and nicotine in Nicotiana sylvestris. Planta 201:397–404. doi:10.1007/s004250050082
Bashi E, Fokkema NJ (1977) Environmental factors limiting growth of Sporobolomyces roseus, an antagonist of Cochliobolus sativus, on wheat leaves. Trans Br Mycol Soc 68(1):17–25. doi:10.1016/S0007-1536(77)80146-0
Bessire M, Chassot C, Jacquat AC, Humphry M, Borel S, Petetot JM, Metraux JP, Nawrath C (2007) A permeable cuticle in Arabidopsis leads to a strong resistance to Botrytis cinerea. EMBO J 26(8):2158–2168. doi:10.1038/sj.emboj.7601658
Buda GJ, Isaacson T, Matas AJ, Paolillo DJ, Rose JK (2009) Three-dimensional imaging of plant cuticle architecture using confocal scanning laser microscopy. Plant J 60(2):378–385. doi:10.1111/j.1365-313X.2009.03960.x
Buschhaus C, Jetter R (2012) Composition and physiological function of the wax layers coating Arabidopsis leaves: β-amyrin negatively affects the intracuticular water barrier. Plant Physiol 160(2):1120–1129. doi:10.1104/pp. 112.198473
Chassot C, Metraux J (2005) The cuticle as source of signals for plant defense. Plant Biosyst 139(1):28–31. doi:10.1080/112635000500056344
Chen S, Su LQ, Chen J, Wu J (2013) Cutinase: characteristics, preparation, and application. Biotechnol Adv 31(8):1754–1767. doi:10.1016/j.biotechadv.2013.09.005
Cominelli E, Galbiati M, Tonelli C (2008) Integration of water stress response: cell expansion and cuticle deposition in Arabidopsis thaliana. Plant Signal Behav 3(8):556–557. doi:10.1111/j.1365-313X.2007.03310.x
Finkelstein R (2013) Abscisic acid synthesis and response. The Arabidopsis Book 11:e0166. doi:10.1199/tab.0166
Fonseca ÁI (2006) Phylloplane yeasts. In: Rosa CAPG (ed) Biodiversity and ecophysiology of yeasts Springer. London, Berlin, pp 264–301
Franke R, Briesen I, Wojciechowski T, Faust A, Yephremov A, Nawrath C, Schreiber L (2005) Apoplastic polyesters in Arabidopsis surface tissues—a typical suberin and a particular cutin. Phytochemistry 66(22):2643–2658. doi:10.1016/j.phytochem.2005.09.027
Holm HC, Nielsen PM (2012) Esterification process. WO2012130961
Ichihara K, Fukubayashi Y (2009) Preparation of fatty acid methyl esters for gas-liquid chromatography. J Lipid Res 51(3):635–640. doi:10.1194/jlr.D001065
Isaacson T, Kosma DK, Matas AJ, Buda GJ, He Y, Yu B, Pravitasari A, Batteas JD, Stark RE, Jenks MA, Rose JK (2009) Cutin deficiency in the tomato fruit cuticle consistently affects resistance to microbial infection and biomechanical properties, but not transpirational water loss. Plant J 60(2):363–377. doi:10.1111/j.1365-313X.2009.03969.x
Ishii M (1988) Positionally non-specific lipase from Candida sp., a method for producing it, its use and a recombinant DNA process for produce it. WO 88/02775
Iuchi S, Kobayashi M, Taji T, Naramoto M, Seki M, Kato T, Tabata S, Kakubari Y, Yamaguchi-Shinozaki K, Shinozaki K (2001) Regulation of drought tolerance by gene manipulation of 9-cis-epoxycarotenoid dioxygenase, a key enzyme in abscisic acid biosynthesis in Arabidopsis. Plant J 27(4):325–333. doi:10.1046/j.1365-313x.2001.01096.x
Karpushova A, Brummer F, Barth S, Lange S, Schmid RD (2005) Cloning, recombinant expression and biochemical characterisation of novel esterases from Bacillus sp. associated with the marine sponge Aplysina aerophoba. Appl Microbiol Biotechnol 67(1):59–69. doi:10.1007/s00253-004-1780-6
Kitamoto HK, Shinozaki Y, Cao XH, Morita T, Konishi M, Tago K, Kajiwara H, Koitabashi M, Yoshida S, Watanabe T, Sameshima-Yamashita Y, Nakajima-Kambe T, Tsushima S (2011) Phyllosphere yeasts rapidly break down biodegradable plastics. AMB Express 1:44. doi:10.1186/2191-0855-1-44
Kmieciak M, Simpson CG, Lewandowska D, Brown JW, Jarmolowski A (2002) Cloning and characterization of two subunits of Arabidopsis thaliana nuclear cap-binding complex. Gene 283(1–2):171–183. doi:10.1016/S0378-1119(01)00859-9
Kolattukudy PE (1980) Biopolyester membranes of plants: cutin and suberin. Science 208(4447):990–1000. doi:10.1126/science.208.4447.990
Kurtzman C, Fell J, Boekhout T (2011) The yeasts: a taxonomic study, 5th ed./edited by Cletus P. Kurtzman, Jack W. Fell, and Teun Boekhout edn. Elsevier, New York: Oxford
Laemmli UK (1970) Cleavage of structural proteins during the assembly of the head of bacteriophage T4. Nature 227(5259):680–685. doi:10.1038/227680a0
L'Haridon F, Besson-Bard A, Binda M, Serrano M, Abou-Mansour E, Balet F, Schoonbeek HJ, Hess S, Mir R, Leon J, Lamotte O, Metraux JP (2011) A permeable cuticle is associated with the release of reactive oxygen species and induction of innate immunity. PLoS Pathog 7(7):e1002148. doi:10.1371/journal.ppat.1002148
Lindow SE, Brandl MT (2003) Microbiology of the phyllosphere. Appl Environ Microbiol 69(4):1875–1883. doi:10.1128/AEM. 69.4.1875-1883.2003
Nadakuduti SS, Pollard M, Kosma DK, Allen C Jr, Ohlrogge JB, Barry CS (2012) Pleiotropic phenotypes of the sticky peel mutant provide new insight into the role of CUTIN DEFICIENT2 in epidermal cell function in tomato. Plant Physiol 159(3):945–960. doi:10.1104/pp. 112.198374
Nielsen TB, Ishii M, Kirke O (1999) Lipases A and B from the yeast Candida antarctica. In: Margesin RaS F (ed) Biotechnological applications of cold-adapted organisms. Springer, Berlin Heidelberg, pp 49–61
Nitsch LM, Oplaat C, Feron R, Ma Q, Wolters-Arts M, Hedden P, Mariani C, Vriezen WH (2009) Abscisic acid levels in tomato ovaries are regulated by LeNCED1 and SlCYP707A1. Planta 229(6):1335–1346. doi:10.1007/s00425-009-0913-7
Park JJ, Jin P, Yoon J, Yang JI, Jeong HJ, Ranathunge K, Schreiber L, Franke R, Lee IJ, An G (2010) Mutation in Wilted Dwarf and Lethal 1 (WDL1) causes abnormal cuticle formation and rapid water loss in rice. Plant Mol Biol 74(1–2):91–103. doi:10.1007/s11103-010-9656-x
Patkar S, Bjorkling F, Zundell M, Schulein M, Svendsen A, Hansen HH, Gormsen E (1993) Purification of two lipases from Candida antarctica and their inhibition by various inhibitors. Indian J Chem 32B:76–80
Riederer M, Schonherr J (1986) Thermodynamic analysis of nonelectrolyte sorption in plant cuticles: the effects of concentration and temperature on sorption of 4-nitrophenol. Planta 169(1):69–80. doi:10.1007/BF01369777
Saito S, Hirai N, Matsumoto C, Ohigashi H, Ohta D, Sakata K, Mizutani M (2004) Arabidopsis CYP707As encode (+)-abscisic acid 8′-hydroxylase, a key enzyme in the oxidative catabolism of abscisic acid. Plant Physiol 134(4):1439–1449. doi:10.1104/pp. 103.037614
Schonherr J (1976) Water permeability of isolated cuticular membranes: the effect of pH and cations on diffusion, hydrodynamic permeability and size of polar pores in the cutin matrix. Planta 128(2):113–126. doi:10.1007/BF00390312
Seo S, Okamoto M, Seto H, Ishizuka K, Sano H, Ohashi Y (1995) Tobacco MAP kinase: a possible mediator in wound signal transduction pathways. Science 270(5244):1988–1992. doi:10.1126/science.270.5244.1988
Shinozaki Y, Morita T, Cao XH, Yoshida S, Koitabashi M, Watanabe T, Suzuki K, Sameshima-Yamashita Y, Nakajima-Kambe T, Fujii T, Kitamoto HK (2013) Biodegradable plastic-degrading enzyme from Pseudozyma antarctica: cloning, sequencing, and characterization. Appl Microbiol Biotechnol 97(7):2951–2959. doi:10.1007/s00253-012-4188-8
Sieber P, Schorderet M, Ryser U, Buchala A, Kolattukudy P, Metraux JP, Nawrath C (2000) Transgenic Arabidopsis plants expressing a fungal cutinase show alterations in the structure and properties of the cuticle and postgenital organ fusions. Plant Cell 12(5):721–737. doi:10.1105/Tpc.12.5.721
Tabata J, Narai Y, Sawamura N, Hiradate S, Sugie H (2012) A new class of mealybug pheromones: a hemiterpene ester in the sex pheromone of Crisicoccus matsumotoi. Naturwissenschaften 99(7):567–574. doi:10.1007/s00114-012-0935-z
Watanabe T, Shinozaki Y, Yoshida S, Koitabashi M, Sameshima-Yamashita Y, Fujii T, Fukuoka T, Kitamoto HK (2014) Xylose induces the phyllosphere yeast Pseudozyma antarctica to produce a cutinase-like enzyme which efficiently degrades biodegradable plastics. J Biosci Bioeng 117(3):325–329. doi:10.1016/j.jbiosc.2013.09.002
Yeats TH, Rose JK (2013) The formation and function of plant cuticles. Plant Physiol 163(1):5–20. doi:10.1104/pp. 113.222737
Acknowledgments
We thank D. H. Kaku for technical advise, Dr. E. Suto for the valuable comments, and Ms. X. Cao for technical assistance in this research. This research was financially supported by the National Institute for Agro-Environmental Sciences, Japan, and Science and Technology Research Promotion Program for Agriculture, Forestry, Fisheries and Food Industry.
Author information
Authors and Affiliations
Corresponding author
Rights and permissions
About this article
Cite this article
Ueda, H., Mitsuhara, I., Tabata, J. et al. Extracellular esterases of phylloplane yeast Pseudozyma antarctica induce defect on cuticle layer structure and water-holding ability of plant leaves. Appl Microbiol Biotechnol 99, 6405–6415 (2015). https://doi.org/10.1007/s00253-015-6523-3
Received:
Revised:
Accepted:
Published:
Issue Date:
DOI: https://doi.org/10.1007/s00253-015-6523-3