Abstract
Ocean acidification, a reduction in the pH of the oceans caused by increasing CO2, can have negative physiological effects on marine species. In this study, we examined how CO2-driven acidification affected the growth and survival of juvenile golden king crab (Lithodes aequispinus), an important fishery species in Alaska. Juveniles were reared from larvae in surface ambient pH seawater at the Kodiak Laboratory. Newly molted early benthic instar crabs were randomly assigned to one of three pH treatments: (1) surface ambient pH ~ 8.2, (2) likely in situ ambient pH 7.8, and (3) pH 7.5. Thirty crabs were held in individual cells in each treatment for 127 days and checked daily for molting or death. Molts and dead crabs were photographed under a microscope and measured using image analysis to assess growth and morphology. Mortality was primarily associated with molting in all treatments, differed among all treatments, and was highest at pH 7.5 and lowest at ambient pH. Crabs at pH 7.5 were smaller than crabs at ambient pH at the end of the experiment, both in terms of carapace length and wet mass; had a smaller growth increment after molting; had a longer intermolt period. Carapace morphology was not affected by pH treatment. Decreased growth and increased mortality in laboratory experiments suggest that lower pH could affect golden king crab stocks and fisheries. Future work should examine if larval rearing conditions affect the juvenile response to low pH.
Similar content being viewed by others
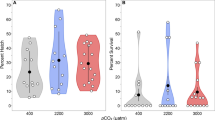
Explore related subjects
Discover the latest articles, news and stories from top researchers in related subjects.Avoid common mistakes on your manuscript.
Introduction
Anthropogenic CO2 is being released into the atmosphere and oceanic uptake of this CO2 has resulted in a decrease of 0.1 pH in global mean surface waters over the last century (Caldeira and Wickett 2003). This reduction in oceanic pH, known as ocean acidification (OA), is predicted to reduce global ocean surface pH to ~ 7.8 by the end of the century and pH ~ 7.5 by the end of 2200 (Caldeira and Wickett 2003; Bopp et al. 2013; IPCC 2014). The rate of pH change is expected to be higher in high-latitude areas, including Alaska waters, in part because CO2 uptake is higher in cold waters (Fabry et al. 2009). Within 50 years, waters around Alaska are predicted to be perennially undersaturated with regard to aragonite (Mathis et al. 2015).
Ocean acidification has a negative effect on many marine species, although the effects are highly variable among taxa, species, and even life history stages (Kroeker et al. 2013). Within the decapod crustaceans, there is a similar range of responses; ocean acidification has a wide range of effects on a number of physiological parameters and can increase mortality (Menu-Courey et al. 2019; Turra et al. 2020), decrease growth (Swiney et al. 2017; McLean et al. 2018), cause morphological deformities (Kurihara et al. 2008; Agnalt et al. 2013), change the metabolic rate (Hans et al. 2014; Long et al. 2019), decrease reproductive output (Swiney et al. 2016), change exoskeleton properties (Coffey et al. 2017), and alter hemolymph chemistry in many species (Pane and Barry 2007), although some species are quite tolerant (deVries et al. 2016; Glandon and Miller 2017; Glandon et al. 2019). This is of concern, both because crustaceans are an important part of many ecosystems (Fabry et al. 2008) and because many species support high-value fisheries, and OA is projected to decrease the stock size and fishery yields (Punt et al. 2016, 2020; Heinrich and Krause 2017).
Golden king crab (Lithodes aequispinus) is an ecologically important deep-water species with a distribution range from Japan to British Columbia, Canada. In Alaska, they are a commercially important species found in the Gulf of Alaska, Bering Sea, and Aleutian Island waters (Donaldson and Byersdorfer 2005); the Aleutian Islands golden king crab fishery had an ex-vessel gross revenue of $23.74 million in 2012 (Garber-Yonts and Lee 2013). Golden king crab are found approximately 200 to 1000 m depth (Donaldson and Byersdorfer 2005), but data on the depth distribution are non-existent for both larvae and newly settled young-of-the-year crab. Older juveniles, sub-mature but CL > ~ 40 mm, are found on continental slopes between 350 and 400 m depth in the Sea of Okhotsk, at approximately 500 m depth in the Bering Sea (Tarverdieva and Zgurovsky 1985), at depths greater than 548 m in the eastern Aleutian Islands (Blau et al. 1996), and between 623 and 583 m depth on the Patton Seamount in the Gulf of Alaska (Shirley 2006). Shirley and Zhou (1997) hypothesized that golden king crab larvae occur at depths greater than 200 m and remain near the benthic substrate; if this is true, then juvenile settlement likely occurs at these depths. In contrast, juveniles in northern British Columbia fjords are most commonly found in waters less than 100 m deep (Sloan 1985).
It is important to examine the effects of ocean acidification on different life stages of the golden king crab since most if not all stages of this deep-water species are likely already living in water at a pH well below surface oceanic pHs. Most carbon chemistry data are limited to surface, shallow, and shelf waters, not the deep-waters that golden king crab inhabit; we summarize the available information in the terms used in the studies cited, but do not mean to imply that any one measure of the carbonate system (e.g., pH or saturation state) is necessarily the driving factor in the crabs’ physiological response. However, the current calcite saturation horizon is approximately 250 m in the eastern Bering Sea (Cross et al. 2013), and in September 2008 in the Gulf of Alaska, waters deeper than approximately 175 to 225 m were undersaturated with regard to aragonite (Fabry et al. 2009). These calcite and aragonite saturation horizons are shallower than the depths that golden king crab of all life stages are generally thought to inhabit (Donaldson and Byersdorfer 2005). In the Gulf of Alaska, the pH at the depths that adult and older juvenile golden king crab inhabit are between about pH 7.7 and 7.8 (Byrne et al. 2010). In this study, we reared young-of-the-year golden king crab in surface ambient pH (~ 8.2, pCO2 ~ 325 ppm), pH 7.8 (likely in situ pH, pCO2 ~ 800 ppm), and pH 7.5 (pCO2 ~ 1600 ppm) waters for 127 days to study the effects of ocean acidification on survival, growth, and morphology.
Methods
Sample collection and laboratory study
Ovigerous golden king crab were collected from the Aleutian Islands, Alaska (51°18.30′N, 179°2.49′E and 52°17.47′N, 175°14.02′E), March and May 2013, and shipped live to the Alaska Fisheries Science Center’s Kodiak Laboratory seawater facility in Kodiak, Alaska, where larval rearing took place from December 2013 to March 2014. Larvae from two females were reared in surface ambient pH flow-through water (temperatures 4–5 °C, Salinity ~ 31, pH ~ 8.0) and, because the larvae are lecithotrophic, were unfed. The subsequent young-of-the-year crab were used in this study. Juveniles were approximately 3 weeks after the molt to C1 and were likely all at the C1 or C2 stage at the beginning of the experiment.
Ninety young-of-the-year crab were randomly assigned to one of three acidification treatments based on projected global ocean surface pH levels: (1) surface ambient (hereafter ambient) pH ~ 8.2, (2) pH 7.8 (c. ~ 2100), and (3) pH 7.5 (c. ~ 2200) (Caldeira and Wickett 2003), for a total of 30 young-of-the-year crab per treatment. The surface ambient treatment represents local ambient conditions, not the typical conditions for young-of-the-year golden king crab; the pH 7.8 treatment is reflective of the in situ pH in older juvenile golden king crab habitat and is likely similar to that of younger juveniles (Byrne et al. 2010); the pH 7.5 treatment is acidified relative to both the current surface ambient and in situ golden king crab conditions. Throughout this paper we refer to pH because it is the parameter we were controlling for; however, we recognize that pH is not necessarily the only or even main driver of the physiological response of marine animals among the component of the carbonate chemistry of seawater. In this experiment, because temperature, pressure, and salinity were all constant, by keeping the pH within a narrow range we also kept each of the other components of the carbonate system within a narrow range (Table 1). Each treatment was contained in a 53 (L) × 38 (W) × 23 (H) cm tub that was placed randomly in the experimental area. Each tub had a flow rate of ~ 250 ml/minute and water was chilled to 5 °C (Table 1). Although there was no replication at the tub level, given the isolation of the crabs from each other combined with a high flow rate, the effect of tub can reasonably be assumed to be negligible. A temperature logger was placed into each tank and data recorded every 30 min. Tubs were covered with 88.9 µm thick black plastic to minimize light penetration. Young-of-the-year crab were reared in individual inserts constructed from PVC pipe 40 mm inner diameter with 750 µm mesh attached to the bottom, and the inserts were placed inside the treatment tub. This size insert was determined to be the optimal size for individual rearing of juvenile red king crab larger than the crab in this study (Swiney et al. 2013); therefore, it was assumed that this size insert was more than adequate for this study. Inserts were raised off the bottom of the tub by placing them on plastic grating. Water was delivered into each insert via a submersible pump connected to a manifold. Crab were fed three times per week to excess on a gel diet of Gelly Belly (Florida Aqua Farms, Inc., Dade City, FL, USA) enhanced with Cyclop-eeze powder (Argent Laboratories, Redmond, WA, USA), pollock bone powder (US Department of Agriculture, Agricultural Research Service, Kodiak, AK, USA), and astaxanthin. Old food was removed prior to feeding.
Each insert was checked daily for molts and mortalities, and exuvia and mortalities were removed for growth and morphometric analysis. At the end of the experiment, the remaining crab were carefully blotted dry with a tissue, weighed, and placed in a − 80 °C freezer. Carapaces from exuvia, mortalities, and from the live crabs at end of the experiment were photographed under a dissecting microscope. Carapace width, carapace length, rostrum base width, orbital spine width, and the first spine length (Fig. 1) were measured using Image Pro Plus v. 7.0.1.658 imaging software (Media Cybernetics, Inc., Bethesda, MD, USA) (Long et al. 2013b). All these measurements were included in the morphometric analysis (below). Throughout this paper “initial” refers to the size of the crabs at the beginning of the experiment, “final” refers to the size of surviving crabs at the end of the experiment, and “1st molt” to the size of crabs after molting the first time. No crabs molted more than once. The experiment began on April 15, 2014, and ran for 127 days.
Seawater acidification
Sand filtered seawater was acidified using the same methods described in Long et al. (2013a). Ambient treatment water was pumped into the laboratory from 15 and 26 m depth intakes. In short, a tank of pH 5.5 was established by bubbling CO2 into ambient seawater. This pH 5.5 water was mixed with ambient seawater in the treatment head tanks via peristaltic pumps controlled by Honeywell controllers and Durafet III pH probes. The ambient head tank did not receive any pH 5.5 water. Waters from the treatment head tanks were then supplied to the treatment tubs. pHF (free scale) and temperature were measured daily in each experimental tub using a Durafet III pH probe calibrated with a TRIS buffer, and when the pH deviated from the target pH by more than ± 0.02 pH units, the Honeywell controller set points were adjusted to bring the pH back to the target value. Weekly water samples from the treatment tubs were taken and poisoned with mercuric chloride unless they were analyzed the same day as collection. Total alkalinity (TA) was determined at the Kodiak Laboratory using a VINDTA 3S (Marianda, Kiel, Germany), and dissolved inorganic carbon (DIC) was determined at an analytic laboratory using a VINDTA 3C coupled to a 5012 Coulometer (UIC Inc., Joliet, IL). Both laboratories used Certified Reference Material from the Dickson Laboratory (Scripps Institute, San Diego, CA) and the procedures in DOE (1994). The other components of the carbonate chemistry in the seawater were calculated using the seacarb package in R (V 3.6.1, Lavigne and Gattuse 2012).
Statistical analysis
Differences in growth, expressed as carapace length (CL), were analyzed with separate ANOVAs for mean initial size, size after molting, and percentage increase in size \((100\frac{{CL}_{initial}-{CL}_{final}}{{CL}_{initial}}\)) in Systat (v13.2.01, San Jose, CA). The number of days from the beginning of the experiment until molting was also analyzed with an ANOVA. Wet weight at the end of the experiment for crab that had all of their limbs was analyzed with a fully crossed ANOVA. Carapace length and percentage increase in size or wet weight were the dependent variables and pH the factor. When significant differences were detected, Tukey’s HSD post hoc pairwise comparison was run to examine differences among treatments. Levene’s test for homogeneity of variance and the Anderson–Darling test for normality were used to determine if data met the assumptions of ANOVA; data were found to meet the assumptions, except initial size was not normally distributed.
Morphometric measurements were normalized (expressed in terms of their z values) prior to analysis to ensure that measurements of larger features did not dominate the analyses. Morphometrics were analyzed with a fully crossed two-way permutational analysis of variance (PERMANOVA) with pH treatment and molt number (initial measurements and measurements taken after the 1st molt) as factors in Primer 6.1.13 (Plymouth, UK). The assumption of homogeneity of dispersion was verified with the permutational dispersion test. The data were visualized using a nonmetric multidimensional plot.
Survival in each treatment was fit to a set of models using maximum likelihood and assuming a binomial distribution of errors in R. In the first set of models developed a priori, we assumed a constant mortality rate such that:\({p}_{m}={e}^{-mt}\), where pm is the cumulative probability of mortality, m is the mortality rate and t is the time in days. We included models where m was constant among the treatments and where it differed among the three treatments. However, these models proved a very poor fit to the data based on visual assessments of the predictions compared to the data; on closer examination, it appeared that mortality increased substantially in the latter part of the experiment at about the same time that molting was occurring. Since molting was relatively synchronous within each treatment (as expected given the crabs were all from the same batch of larvae), this led us to include logistic (sigmoidal) models such that \({p}_{m}=s+\frac{1-s}{1+{(\frac{t}{{t}_{50}})}^{b}}\) , where s is the predicted final survival, t50 is the time in days to 50% mortality, and b is a slope parameter. We fit two logistic models to the data, one in which the three parameters were the same among the treatments and one in which they all differed. Although these models were an improvement over the constant mortality models, visual assessments of the fits indicated they too were unsatisfactory, in part because they failed to capture mortality at times other than molting. To accommodate mortality during both molting and during the intermolt, we considered a series of models where the mortality rate was allowed to vary over time such that \({S}_{t}={S}_{t-1}(1-{m}_{t})\) where St is the cumulative survival at time = t and mt is the daily mortality rate at time = t. Note that when mt is constant for all t the model is identical to the constant survival model above. We then modeled mt as a combination of base, constant mortality and mortality during molting such that \({m}_{t}=m+{E}_{t}{m}_{molt}\) where m is the base mortality rate for all causes except molting, Et is the probability of molting (ecdysis) at time = t, and mmolt is the probability of death during molting. As above, because molting was synchronous, we considered that the cumulative probability of molting could be modeled as a logistic equation \({p}_{molt}=\frac{1}{1+{(\frac{t}{{t}_{molt50}})}^{b}}\), where tmolt50 is the time at which 50% of the crabs had molted. The rate of molting is then proportional to the slope of this logistic curve such that \({E}_{t}=c\frac{d{p}_{molt}}{dt}=c\frac{b{t}_{molt50}^{b}{t}^{b-1}}{{({t}^{b}+{t}_{molt50}^{b})}^{2}},\) where c is a constant of proportionality. Thus, \({m}_{t}=m+c{m}_{molt}\frac{b{t}_{molt50}^{b}{t}^{b-1}}{{({t}^{b}+{t}_{molt50}^{b})}^{2}}\). In this model c and mmolt cannot be estimated independently so we substituted in a combined constant d such that \(d=c{m}_{molt}\). We then fit three models, one where the three pH treatments were the same, one in which m was constant among the three pH treatments and b, tmolt50, and d varied, and one where m, b, tmolt50, and d all varied among the pH treatments. We calculated the Akaike's Information Criterion corrected for sample size (AICc) for each model and used it to select the best one. Models with a ΔAICc of < 2 were considered to explain the data equally well (Burnham and Anderson 2002).
Results
The data and metadata underlying this article are included as a supplement (Online Resource 1).
Target temperature and pHs were achieved through the experiment. DIC increased with decreasing pH, pCO2 increased with decreasing pH, and alkalinity did not vary with treatment. Aragonite was supersaturated in the ambient treatment but undersaturated in the pH 7.8 and pH 7.5 treatments. Calcite was supersaturated in the ambient and pH 7.8 treatments, and undersaturated in the pH 7.5 treatment (Table 1).
At the end of the experiment, all crabs that had not died had molted once. Overall 17 crabs successfully molted in the ambient treatment, 20 in pH 7.8, and 14 in pH 7.5. Young-of-the-year crab golden king crab mean initial size was 2.68 (SE = 0.009) mm CL and did not differ with pH treatment (ANOVA, F2,74 = 0.290, p = 0.749), but after molting CL differed with treatment (ANOVA, F2,46 = 4.742, p = 0.013; Fig. 2). Crab from the pH 7.5 treatment were on average smaller than crab from the ambient treatment (Tukey’s HSD, p = 0.010); average size did not differ among the other treatments (Fig. 2). On average, crab CL increased by 6.0% (SE = 0.614) after molting, and the percent increase varied with pH treatment (ANOVA, F2,43 = 4.690, p = 0014; Fig. 3). The average percent increase in CL was higher for the ambient treatment than the pH 7.8 (Tukey’s HSD, p = 0.031) and pH 7.5 (Tukey’s HSD, p = 0.027) treatments, which did not differ from each other (Fig. 3). Crabs in pH 7.5 water took longer to molt than those in ambient or pH 7.8 water (ANOVA, F2,48 = 16.189, p < 0.0005; Fig. 3). Average wet weight at the end of the experiment also differed with treatment (ANOVA, F2,20 = 7.978, p = 0.003); on average, pH 7.5 treatment crab weighed less than ambient (Tukey’s HSD, p = 0.027) and pH 7.8 (Tukey’s HSD, p = 0.002) treatment crab, which did not differ from each other (Fig. 4).
Morphology of golden king crab juveniles did not vary with pH treatment (Pseudo-F2,73 = 0.686, p = 0.697) but did with molt number (Pseudo-F1,73 = 15.388, p < 0.00005) and with their interaction (Pseudo-F2,73 = 2.243, p = 0.030). Post hoc PERMANOVA pairwise comparisons indicated that the morphology of both the ambient and pH 7.8 crabs changed between the initial morphology and after the first molt (ambient: t = 4.074, p < 0.00005, pH 7.8: t = 2.660, p = 0.0004), but this was not true of the pH 7.5 crabs (t = 1.237, p = 0.194; Fig. 5). Despite this, there were no differences in morphology among the pH treatment groups either initially or after the first molt (p > 0.10 in all cases).
Non-metric multidimensional scaling plot of juvenile golden king crab morphometric measurements (see Fig. 1) for crabs held in three pH treatments. “Initial” represents the crabs when first placed into the treatments and “1st molt” represents measurements made after the first molt
In the best-fit model of mortality, mortality was treated as a combination of baseline mortality (i.e., not associated with molting), which was constant over time, and mortality during molting. Both types of mortality differed among pH treatments; no other models had any support at all (Table 2). In all three treatments, there was a low baseline mortality rate throughout the experiment with a substantial increase in mortality rate during the time molting was occurring (Fig. 6). Both baseline mortality and the mortality during molting were lower in the ambient treatment than in the pH 7.8 and pH 7.5 treatment. Baseline mortality was similar between pH 7.8 and pH 7.5, but mortality during molting was higher at pH 7.5 (Fig. 5, Table 2). There was a high degree of correspondence between successfully molting and mortality risk; in the ambient and pH 7.8 treatment no crab that successfully molted subsequently died and in the pH 7.5 only three crabs that successfully molted later died. In addition, of the 60 crabs that died, 12 died while molting. The sigmoidal models predicted peak mortality a little after the peak in molting in all three treatments (Fig. 6). At the end of the experiment, there were 12 crabs surviving in the ambient treatment, 8 in the pH 7.8, and 8 in the pH 7.5.
a Percent of initial number young-of-the-year golden king crab surviving on each day by pH treatment and best-fit model (model m (T), mmolt (T), Table 2). Stepwise lines represent observed survival and smoothed lines predicted survival. b Daily mortality rates predicted by the best-fit model for each pH treatment. Horizontal lines represent the average days to molt for each of the pH treatments
Discussion
Juvenile golden king crabs exposed to pH levels below surface ambient had significantly lower growth and survival than those exposed to surface ambient water. These results suggest that population dynamics of golden king crab could be affected by ocean acidification within this century and are similar to the effects of ocean acidification on other species of king crab in the Bering Sea (Long et al. 2013b, 2017). Higher mortality and slower growth would decrease population abundance and productivity and have a corresponding effect on the directed fisheries.
Juvenile golden king crab showed decreased growth under low pH conditions. Time to first molt was increased by 34% in pH 7.5 compared to surface ambient pH and the growth increment was 49 and 45% lower in pH 7.5 and 7.8 compared to surface ambient. Overall, this resulted in crabs that were larger in the surface ambient treatment than in the other two by the end of the experiment. This is similar to what has been observed in other lithodid species; both red and blue king crabs (Paralithodes camtschaticus and P. platypus) have slower growth under reduced pH (Long et al. 2013b, 2017). Likewise, other crustacean species, such as the American lobster (Homarus americanus), exhibit decreased growth under ocean acidification (Small et al. 2016; McLean et al. 2018). In golden king crab, however, the change in growth was not accompanied by changes in morphology. Both H. americanus and the shrimp Palaemon pacificus exhibit deformities under low pH (Kurihara et al. 2008; Agnalt et al. 2013), suggesting that low pH can interfere with cuticle formation or hardening. Similarly, red and blue king crab did not exhibit any morphological change associated with pH (Long et al. 2013b, 2017), although the micromechanical properties of their exoskeletons were affected (Coffey et al. 2017).
Decreased growth could have several effects on the population or ecological scale. Slower growth would either increase the time it takes for crabs to reach maturity or decrease the size-at-maturity. The longer it takes to achieve maturity the more likely a crab is to die prior to that, and a decrease size-at-maturity would decrease fecundity. This would likely correspond with lower stock productivity. Further, for most species of crabs, smaller crabs are more vulnerable to predation. Red king crab juveniles have a decreasing chance of being predated upon as they get larger (Pirtle et al. 2012; Long et al. 2018), and juvenile blue crab, Callinecetes sapidus, suffer high predatory mortality when small, but get a partial size refute from predation once they reach 40 mm carapace width (Johnson et al. 2008). Thus, decrease growth could indirectly increase predatory mortality for juvenile crabs.
Mortality of golden king crab juveniles was increased at both pH 7.8 and 7.5. This is a common response among crustaceans; red and blue king crab (Paralithodes camtschaticus and P. platypus), Tanner crab (Chionoecetes bairdi), the European lobster (Homarus gammarus), and the shrimps Metapenaeus joyneri and Palaemon pacificus all suffer increased mortality under acidified conditions (Kurihara et al. 2008; Dissanayake and Ishimatsu 2011; Long et al. 2013b, 2017; Small et al. 2016). The best-fit model of mortality split the mortality between molting and all other sources and was an excellent fit to the data (as estimated visually, Fig. 6). Both sources of mortality were increased under reduced pH. For golden king crab, the majority of the mortality in all treatments was associated with molting. The peak in mortality rate in all three treatments occurred just slightly after the average time-to-molt (Fig. 6, Table 2), suggesting that many of the crabs which died were those that did not molt successfully, and many crabs died while trying to molt. Molting is a physiologically complicated and energetically expensive process in crustaceans (Roberts 1957; Mangum et al. 1985; Chang 1995), so it is not surprising to see elevated mortality prior to molting under physiologically stressful conditions. A similar association between mortality and molting under acidified conditions occurs in juvenile H. gammarus, mature female red king crab, and adult M. joyneri (Dissanayake and Ishimatsu 2011; Long et al. 2013a; Small et al. 2016).
The decreased growth and increased mortality in golden king crab in acidified waters suggest that the crabs are responding physiologically to the decreased pH. Most decapod species respond to increased pCO2 by increasing bicarbonate transport into the hemolymph, which in turn reduces or completely eliminates the change in hemolymph pH (e.g., Pane and Barry 2007; Appelhans et al. 2012; Knapp et al. 2016). This active transport is likely energetically expensive; juvenile red and blue king crabs both greatly increase respiration rates immediately after exposure to acidified water: 73% for red king crab and 178% for blue (Long et al. 2019). If the energetic costs of maintaining acid–base homeostasis are higher under reduced pH conditions, then that would leave less energy available for other biological functions, such as growth, and indirectly cause an increased mortality rate during energetically expensive life-history events such as molting. Alternatively, as is the case for other deepwater species (Pane and Barry 2007), golden king crab may not be able to regulate their hemolymph pH or may only be able to partially compensate for reduced environmental pH. Altered hemolymph and intracellular pH would have a suite of physiological consequences including decrease enzymatic actives (Tanner et al. 2006) which would also explain the decreased growth and increased mortality observed.
That juvenile golden king crab should be negatively affected at a pH they are known to live at naturally is a surprising result; one would presume that the animals are well adapted to those conditions. There are several non-exclusive possibilities that may explain these patterns. It is possible that the growth and mortality rates that we observed at pH 7.8 are reflective of what actually occurs in situ. This is possible, but it seems unlikely that a species would not be better adapted to its local environment. Alternatively, it could be that laboratory rearing of larvae at surface water pH resulted in juveniles that were physiologically adapted or acclimated to surface pH, either through the selection of individuals adapted to surface pH, phenotypic plasticity induced at the larval stage, or a combination of these mechanisms. Species including the Sydney rock oyster (Saccostrea glomerata), the calanoid copepod (Pseudocalanus acuspes), and the Manila clam (Ruditapes philippinarum) show high evolutionary potential in selective breeding and transgenerational experiments (Parker et al. 2012, 2015; Thor and Dupont 2015; Zhao et al. 2018), although other species do not (Langer et al. 2019).
We hypothesize, therefore, that golden king crab have a potential for acclimatization or adaptation to a range of pH conditions at least within the range of pHs that occur along their depth distribution; though this may not extend to lower pHs. Although juveniles in the Aleutian Islands population of golden king crab, where the crabs in this study came from, occur at depths greater than 500 m (Blau et al. 1996), populations in British Columbia fjords occur at depths from 50 to 400 m (Sloan 1985). Given that pH is highly depth dependent in such systems, this suggests there is wide variance in golden king crab tolerance for a range of pH conditions both with and among populations.
Future work should focus on examination of carryover effects on juveniles from the embryo and larval stages. If, as hypothesized above, the response of juvenile golden king crab is determined by the pH at the larval stage, a fully crossed experiment would test this effectively. Further, a comparison of gene expression and hemolymph chemistry among the treatments could help to pinpoint the underlying biochemical mechanisms. Finally, understanding the interactive effect of other potential co-stressors, including increased temperature and low dissolved oxygen is essential for this deep-dwelling, cold-water species (Breitburg et al. 2015).
Availability of data and materials
The datasets generated during and/or analyzed during the current study included as a supplement to this paper.
Code availability
The code generated during the current study is available from the corresponding author on reasonable request.
References
Agnalt AL, Grefsrud ES, Farestveit E, Larsen M, Keulder F (2013) Deformities in larvae and juvenile European lobster (Homarus gammarus) exposed to lower pH at two different temperatures. Biogeosciences 10:7883–7895. https://doi.org/10.5194/bg-10-7883-2013
Appelhans YS, Thomsen J, Pansch C, Melzner F, Wahl M (2012) Sour times: seawater acidification effects on growth, feeding behaviour and acid-base status of Asterias rubens and Carcinus maenas. Mar Ecol Progr Ser 459:85–98. https://doi.org/10.3354/meps09697
Blau SF, Pengilly D, Tracy DA (1996) Distribution of golden king crabs by sex, size, and depth zones in the Eastern Aleutian Islands, Alaska. In: Baxter B, Donaldson WE, Paul AJ, Otto RS, Witherell DB (eds) High latitude crabs: biology. Management and Economics Alaska Sea Grant College Program, University of Alaska Fairbanks, Anchorage, AK, pp 167–185
Bopp L, Resplandy L, Orr JC, Doney SC, Dunne JP, Gehlen M, Halloran P, Heinze C, Ilyina T, Seferian R (2013) Multiple stressors of ocean ecosystems in the 21st century: projections with CMIP5 models. Biogeosciences 10:6225–6245. https://doi.org/10.5194/bg-10-6225-2013
Breitburg DL, Salisbury J, Bernhard JM, Cai W-J, Dupont S, Doney SC, Kroeker KJ, Levin LA, Long WC, Milke LM (2015) And on top of all that… Coping with ocean acidification in the midst of many stressors. Oceanography 28:48–61. https://doi.org/10.5670/oceanog.2015.31
Burnham KP, Anderson DR (2002) Model selection and multimodel inference: a practical information-theoretic approach. Springer, New York
Byrne RH, Mecking S, Feely RA, Liu X (2010) Direct observations of basin-wide acidification of the North Pacific Ocean. Geophys Res Lett 37:L02601
Caldeira K, Wickett ME (2003) Anthropogenic carbon and ocean pH. Nature 425:365. https://doi.org/10.1038/425365a
Chang ES (1995) Physiological and biochemical changes during the molt cycle in decapod crustaceans: an overview. J Exp Mar Biol Ecol 193:1–14
Coffey WD, Yarram A, Matoke B, Long WC, Swiney KM, Foy RJ, Dickenson G (2017) Ocean acidification leads to altered micromechanical properties of the mineralized cuticle of juvenile red and blue king crabs. J Exp Mar Biol Ecol 495:1–12. https://doi.org/10.1016/j.jembe.2017.05.011
Cross JN, Mathis JT, Bates NR, Byrne RH (2013) Conservative and non-conservative variations of total alkalinity on the southeastern Bering Sea shelf. Mar Chem 154:100–112. https://doi.org/10.1016/j.marchem.2013.05.012
deVries MS, Webb SJ, Tu J, Cory E, Morgan V, Sah RL, Deheyn DD, Taylor JRA (2016) Stress physiology and weapon integrity of intertidal mantis shrimp under future ocean conditions. Sci Rep 6:15. https://doi.org/10.1038/srep38637
Dissanayake A, Ishimatsu A (2011) Synergistic effects of elevated CO2 and temperature on the metabolic scope and activity in a shallow-water coastal decapod (Metapenaeus joyneri; Crustacea: Penaeidae). ICES J Mar Sci 68:1147–1154. https://doi.org/10.1093/icesjms/fsq188
DOE (1994) Handbook of methods for the analysis of the various parameters of the carbon dioxide system in sea water. Version 2. U.S. Department of Energy
Donaldson W, Byersdorfer S (2005) Biological field techniques for lithodid crabs. University of Alaska Fairbanks, Fairbanks, AK, Alaska Sea Grant College Program
Dupont S, Dorey N, Stumpp M, Melzner F, Thorndyke M (2013) Long-term and trans-life-cycle effects of exposure to ocean acidification in the green sea urchin Strongylocentrotus droebachiensis. Mar Biol 160:1835–1843. https://doi.org/10.1007/s00227-012-1921-x
Fabry VJ, Seibel BA, Feely RA, Orr JC (2008) Impacts of ocean acidification on marine fauna and ecosystem processes. ICES J Mar Sci. https://doi.org/10.1093/icesjms/fsn048
Fabry VJ, McClintock JB, Mathis JT, Grebmeier JM (2009) Ocean acidification at high latitudes: the bellwether. Oceanography 22:160–171
Garber-Yonts B, Lee J (2013) Stock Assessment and Fishery Evaluation Report for King and Tanner Crab Fisheries of the Bering Sea and Aleutian Islands Regions: Economic Status of the BSAI Crab Fisheries, 2013. National Oceanic and Atmospheric Administration, National Marine Fisheries Service, Alaska Fisheries Science Center, Seattle, Washington
Glandon HL, Miller TJ (2017) No effect of high pCO2 on juvenile blue crab, Callinectes sapidus, growth and consumption despite positive responses to concurrent warming. ICES J Mar Sci 74:1201–1209. https://doi.org/10.1093/icesjms/fsw171
Glandon HL, Paynter KT, Rowe CL, Miller TJ (2019) Resilience of oxygen consumption rates in the juvenile blue crab Callinectes sapidus to future predicted increases in environmental temperature and pCO2 in the mesohaline Chesapeake Bay. J Shellfish Res 38:711–723. https://doi.org/10.2983/035.038.0323
Hans S, Fehsenfeld S, Treberg JR, Weihrauch D (2014) Acid-base regulation in the Dungeness crab (Metacarcinus magister). Mar Biol 161:1179–1193. https://doi.org/10.1007/s00227-014-2409-7
Heinrich L, Krause T (2017) Fishing in acid waters: a vulnerability assessment of the Norwegian fishing industry in the face of increasing ocean acidification. Integr Environ Assess Manage 13:778–789. https://doi.org/10.1002/ieam.1843
IPCC (2014) Climate Change 2014 Fifth Assessment Synthesis Report
Johnson EG, Hines AH, Kramer MA, Young AC (2008) Importance of season and size of release to stocking success for the blue crab in Chesapeake Bay. Rev Fish Sci 16:243–253
Kelly MW, Padilla-Gamino JL, Hofmann GE (2013) Natural variation and the capacity to adapt to ocean acidification in the keystone sea urchin Strongylocentrotus purpuratus. Global Chang Biol 19:2536–2546. https://doi.org/10.1111/gcb.12251
Knapp JL, Bridges CR, Krohn J, Hoffman LC, Auerswald L (2016) The effects of hypercapnia on the West Coast rock lobster (Jasus lalandii) through acute exposure to decreased seawater pH—physiological and biochemical responses. J Exp Mar Biol Ecol 476:58–64. https://doi.org/10.1016/j.jembe.2015.12.001
Kroeker KJ, Kordas RL, Crim R, Hendriks IE, Ramajo L, Singh GS, Duarte CM, Gattuso JP (2013) Impacts of ocean acidification on marine organisms: quantifying sensitivities and interaction with warming. Global Chang Biol 19:1884–1896
Kurihara H, Matsui M, Furukawa H, Hayashi M, Ishimatsu A (2008) Long-term effects of predicted future seawater CO2 conditions on the survival and growth of the marine shrimp Palaemon pacificus. J Exp Mar Biol Ecol 367:41–46. https://doi.org/10.1016/j.jembe.2008.08.016
Langer JAF, Meunier CL, Ecker U, Horn HG, Schwenk K, Boersma M (2019) Acclimation and adaptation of the coastal calanoid copepod Acartia tonsa to ocean acidification: a long-term laboratory investigation. Mar Ecol Progr Ser 619:35–51. https://doi.org/10.3354/meps12950
Lavigne H, Gattuse J (2012) seacarb: Seawater carbonate chemistry with R. http://CRAN.R-project.org/package=seacarb
Lee YH, Jeong CB, Wang MH, Hagiwara A, Lee JS (2020) Transgenerational acclimation to changes in ocean acidification in marine invertebrates. Mar Pollut Bull 153:18. https://doi.org/10.1016/j.marpolbul.2020.111006
Long WC, Swiney KM, Foy RJ (2013a) Effects of ocean acidification on the embryos and larvae of red king crab, Paralithodes camtschaticus. Mar Pollut Bull 69:38–47. https://doi.org/10.1016/j.marpolbul.2013.01.011
Long WC, Swiney KM, Harris C, Page HN, Foy RJ (2013b) Effects of ocean acidification on juvenile red king crab (Paralithodes camtschaticus) and Tanner crab (Chionoecetes bairdi) growth, condition, calcification, and survival. PLoS ONE 8:e60959. https://doi.org/10.1371/journal.pone.0060959
Long WC, Van Sant SB, Swiney KM, Foy R (2017) Survival, growth, and morphology of blue king crabs: effect of ocean acidification decreases with exposure time. ICES J Mar Sci 74:1033–1041. https://doi.org/10.1093/icesjms/fsw197
Long WC, Cummiskey P, Munk JE (2018) How does stocking density affect enhancement success for hatchery-reared red king crab? Can J Fish Aquat Sci 75:1940–1948. https://doi.org/10.1139/cjfas-2017-0330
Long WC, Pruisner P, Swiney KM, Foy R (2019) Effects of ocean acidification on respiration, feeding, and growth of juvenile red and blue king crabs (Paralithodes camtschaticus and P. platypus). ICES J Mar Sci 76:1335–1343. https://doi.org/10.1093/icesjms/fsz090
Mangum C, McMahon B, Defur P, Wheatly M (1985) Gas exchange, acid-base balance, and the oxygen supply to the tissues during a molt of the blue crab Callinectes sapidus. J Crust Biol 5:188–206
Mathis JT, Cross JN, Evans W, Doney SC (2015) Ocean acidification in the surface waters of the Pacific-Arctic boundary regions. Oceanography 28:122–135. https://doi.org/10.5670/oceanog.2015.36
McLean EL, Katenka NV, Seibel BA (2018) Decreased growth and increased shell disease in early benthic phase Homarus americanus in response to elevated CO2. Mar Ecol Prog Ser 596:113–126
Menu-Courey K, Noisette F, Piedalue S, Daoud D, Blair T, Blier PU, Azetsu-Scott K, Calosi P (2019) Energy metabolism and survival of the juvenile recruits of the American lobster (Homarus americanus) exposed to a gradient of elevated seawater pCO2. Mar Environ Res 143:111–123. https://doi.org/10.1016/j.marenvres.2018.10.002
Pane EF, Barry JP (2007) Extracellular acid-base regulation during short-term hypercapnia is effective in a shallow-water crab, but ineffective in a deep-sea crab. Mar Ecol Prog Ser 334:1–9. https://doi.org/10.3354/meps334001
Parker LM, Ross PM, O’Connor WA, Borysko L, Raftos DA, Pörtner HO (2012) Adult exposure influences offspring response to ocean acidification in oysters. Global Chang Biol 18:82–92
Parker LM, O’Connor WA, Raftos DA, Pörtner H-O, Ross PM (2015) Persistence of positive carryover effects in the oyster, Saccostrea glomerata, following transgenerational exposure to ocean acidification. PLoS ONE 10:e0132276. https://doi.org/10.1371/journal.pone.0132276
Pirtle JL, Eckert GL, Stoner AW (2012) Habitat structure influences the survival and predator-prey interactions of early juvenile red king crab Paralithodes camtschaticus. Mar Ecol Prog Ser 465:169–184. https://doi.org/10.3354/meps09883
Punt AE, Foy RJ, Dalton MG, Long WC, Swiney KM (2016) Effects of long term exposure to ocean acidification on future southern Tanner crab (Chionoecetes bairdi) fisheries management. ICES J Mar Sci 73:849–864
Punt AE, Dalton MG, Foy RJ (2020) Multispecies yield and profit when exploitation rates vary spatially including the impact on mortality of ocean acidification on North Pacific crab stocks. Fish Res 225:18. https://doi.org/10.1016/j.fishres.2019.105481
Roberts JL (1957) Thermal acclimation of metabolism in the crab Pachygrapsus crassipes Randall. I. The influence of body size, starvation, and molting. Physiol Zool 30:232–242
Shirley TC (2006) Cultivation potential of golden king crab, Lithodes aequispinus. In: Stevens BG (ed) Alaska Crab Stock Enhancement and Rehabilitation. Alaska Sea Grant College Program, Kodiak, Alaska, pp 47–54
Shirley T, Zhou S (1997) Lecithotrophic development of the golden king crab Lithodes aequispinus (Anomura: Lithodidae). J Crust Biol 17:207–216
Sloan N (1985) Life history characteristics of fjord-dwelling golden king crabs Lithodes aequispina. Mar Ecol Progr Ser 22:219–228
Small DP, Calosi P, Boothroyd D, Widdicombe S, Spicer JI (2016) The sensitivity of the early benthic juvenile stage of the European lobster Homarus gammarus (L.) to elevated pCO2 and temperature. Mar Biol 163:1–12
Swiney KM, Long WC, Persselin SL (2013) The effects of holding space on juvenile red king crab (Paralithodes camtschaticus) growth and survival. Aquacult Res 44:1007–1016. https://doi.org/10.1111/j.1365-2109.2012.03105.x
Swiney KM, Long WC, Foy RJ (2016) Effects of high pCO2 on Tanner crab reproduction and early life history- Part I: long-term exposure reduces hatching success and female calcification, and alters embryonic development. ICES J Mar Sci 73: 825–835
Swiney KM, Long WC, Foy RJ (2017) Decreased pH and increased temperatures affect young-of-the-year red king crab (Paralithodes camtschaticus). ICES J Mar Sci 74:1191–1200. https://doi.org/10.1093/icesjms/fsw251
Tanner CA, Burnett LE, Burnett KG (2006) The effects of hypoxia and pH on phenoloxidase activity in the Atlantic blue crab, Callinectes sapidus. Comp Biochem Physiol: Mol Integr Physiol 144:218–223
Tarverdieva MI, Zgurovsky KA (1985) On food composition of the deep-water crab species Lithodes aequispina Benedict and Chionoecetes tanneri Rathbun in the Bering Sea and Okhotsk seas. In: Davis SK, Gaffney F, McCrary J, Paul AJ, Otto RS (eds) Proceedings of the International King Crab Symposium. Alaska Sea Grant College Program, University of Alaska Fairbanks, Anchorage, AK, pp 319–329
Thor P, Dupont S (2015) Transgenerational effects alleviate severe fecundity loss during ocean acidification in a ubiquitous planktonic copepod. Global Chang Biol 21:2261–2271. https://doi.org/10.1111/gcb.12815
Thor P, Bailey A, Dupont S, Calosi P, Soreide JE, De Wit P, Guscelli E, Loubet-Sartrou L, Deichmann IM, Candee MM, Svensen C, King AL, Bellerby RGJ (2018) Contrasting physiological responses to future ocean acidification among Arctic copepod populations. Global Chang Biol 24:E365–E377. https://doi.org/10.1111/gcb.13870
Turra A, Ragagnin MN, McCarthy ID, Fernandez WS (2020) The effect of ocean acidification on the intertidal hermit crab Pagurus criniticornis is not modulated by cheliped amputation and sex. Mar Environ Res 153:10. https://doi.org/10.1016/j.marenvres.2019.104794
Walther K, Anger K, Portner HO (2010) Effects of ocean acidification and warming on the larval development of the spider crab Hyas araneus from different latitudes (54 degrees vs. 79 degrees N). Mar Ecol Progr Ser 417:159–170. https://doi.org/10.3354/meps08807
Zhao LQ, Yang F, Milano S, Han TK, Walliser EO, Schone BR (2018) Transgenerational acclimation to seawater acidification in the Manila clam Ruditapes philippinarum: Preferential uptake of metabolic carbon. Sci Total Environ 627:95–103. https://doi.org/10.1016/j.scitotenv.2018.01.225
Zhao L, Liu B, An W, Deng Y, Lu Y, Liu B, Wang L, Cong Y, Sun X (2019) Assessing the impact of elevated pCO2 within and across generations in a highly invasive fouling mussel (Musculista senhousia). Sci Total Environ 689:322–331. https://doi.org/10.1016/j.scitotenv.2019.06.466
Acknowledgements
This work was funded through the NOAA Ocean Acidification Program. We thank B. Daly for rearing the juvenile crab and staff of the Kodiak Laboratory for help performing the experiments. Previous versions of this paper were improved by comments from E. Fedwa and J. Long and two anonymous reviewers. The findings and conclusions in the paper are those of the authors and do not necessarily represent the views of the National Marine Fisheries Service, NOAA. Reference to trade names or commercial firms does not imply endorsement by the National Marine Fisheries Service, NOAA.
Funding
This work was funded through the NOAA Ocean Acidification Program.
Author information
Authors and Affiliations
Corresponding author
Ethics declarations
Conflict of interest
The authors affirm that they have no conflicts of interest.
Ethics approval
Not applicable.
Consent to participate
Not applicable.
Consent for publication
Not applicable.
Additional information
Responsible Editor: H.-O. Pörtner.
Publisher's Note
Springer Nature remains neutral with regard to jurisdictional claims in published maps and institutional affiliations.
Reviewers: undisclosed experts.
Electronic supplementary material
Below is the link to the electronic supplementary material.
Rights and permissions
About this article
Cite this article
Long, W.C., Swiney, K.M. & Foy, R.J. Effects of ocean acidification on young-of-the-year golden king crab (Lithodes aequispinus) survival and growth. Mar Biol 168, 126 (2021). https://doi.org/10.1007/s00227-021-03930-y
Received:
Accepted:
Published:
DOI: https://doi.org/10.1007/s00227-021-03930-y