Abstract
An aptamer was previously selected against the anaphylactic allergen β-conglutin (β-CBA I), which was subsequently truncated to an 11-mer and the affinity improved by two orders of magnitude. The work reported here details the selection and characterisation of a second aptamer (β-CBA II) selected against a second aptatope on the β-conglutin target. The affinity of this second aptamer was similar to that of the 11-mer, and its affinity was confirmed by three different techniques at three independent laboratories. This β-CBA II aptamer in combination with the previously selected β-CBA I was then exploited to a dual-aptamer approach. The specific and simultaneous binding of the dual aptamer (β-CBA I and β-CBA II) to different sites of β-conglutin was confirmed using both microscale thermophoresis and surface plasmon resonance where β-CBA II serves as the primary capturing aptamer and β-CBA I or the truncated β-CBA I (11-mer) as the secondary signalling aptamer, which can be further exploited in enzyme-linked aptamer assays and aptasensors.
Similar content being viewed by others
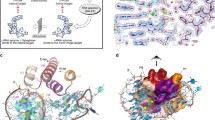
Avoid common mistakes on your manuscript.
Introduction
Antibodies have been exploited in affinity-based detection systems for a wide variety of applications ranging from food quality control and environmental monitoring to clinical diagnostics and are routinely used in different types of enzyme-linked immunosorbent assays (ELISA). Sandwich ELISA is a highly robust assay that uses antibodies recognising two different epitopes on the target molecule and has been highly exploited since its inception for the detection of a wide range of medium-large molecules. Furthermore, recombinant antibodies have been designed to bind to adjacent, non-overlapping epitopes simultaneously, demonstrating higher affinity and specificity [1–3].
Aptamers are nucleic acids that can bind to a wide range of diverse targets, from small molecules to proteins and even cells, with high affinity and specificity due to their specific 3D structure [4]. The process used for the selection and isolation of these aptamers is termed systematic evolution of ligands by exponential enrichment (SELEX) characterised by the repetition of successive steps consisting of selection (binding, partition and elution) and amplification [5, 6].
In comparison to antibodies, aptamers have several advantages as recognition elements. They are chemically synthesised and easily modified for a wide range of applications. The thermostability and high flexibility of aptamers offer the possibility for them to be adapted to different assay formats. The first mention of dual aptamers that bound distinct epitopes of human thrombin was reported in 1997 [7], and thrombin dual aptamers have been used in several analytical techniques and diagnostic applications [8–17]. Whilst the vast majority of reported aptamers are single aptamers against a single aptatope on its cognate target, there are reports of targets with multiple aptamers against various aptatopes. Four individual aptamers have been demonstrated to bind to different binding sites on the adenocarcinoma A549 cell, but these have not yet been used in a combined detection assay [18]. Aptamer couples have also been detailed for cancer cells including aptamers for differentiation of prostate cancer cells and prostate non-cancerous cells, which have been used both for detection [19, 20] and therapy [21], as well as a combination of aptamers against each of the MUC1 and HER2 breast cancer cells [22], with potential for use as screening tools. The same aptamer has been used as both capture (primary) and reporter (secondary) against the cancer-related platelet-derived growth factor in diverse detection strategies [23, 24]. There are a few recent examples of the exploitation of dual aptamers for the detection of Staphylococcus aureus [25] as well as prion proteins, where one aptamer binds to 23–90 of the N-terminal, whilst the other binds to 90–231, part of the β-sheet structure of the PrP [26]. Other examples include dual aptamers against different binding sites of vaspin [27] as well as the VEa5 and Vap7 aptamers selected against diverse aptatopes on the vascular endothelial growth factor (VEGF165) target. In the examples cited, the simultaneous recognition of an analyte by two aptamers generally provides a higher binding affinity and specificity to their target as compared to the use of any of the individual aptamers alone [21, 27, 28]. Moreover, the use of dual aptamers can be easily implemented to a sandwich platform with improved performance due to the specific characteristics of aptamers over the commonly used dual antibody ELISA format [29].
Leguminous plants including peanut, soybean and lupin are typical examples of foods containing a considerable number of potent allergenic proteins [30–33]. The globulins consisting of two major subunits (β- and α-conglutin) and two minor subunits (γ- and δ-conglutin) are represented by the majority of storage proteins found in lupin seeds [34]. Although there is no consensus regarding the allergenic properties of individual lupin conglutins [35, 36], β-conglutin has been reported to be one of the most frequent causes of severe food-associated anaphylaxis [37–39] and in 2008 was identified by the International Union of Immunological Studies as the Lup an 1 allergen [40].
We have previously selected an aptamer against β-conglutin, termed the β-conglutin binding aptamer (β-CBA I), which showed high affinity and specificity to the target [41]. This highly specific aptamer was used to develop a competitive Apta-PCR assay achieving a detection limit of 8 × 10−11 M [42]. β-CBA I was subsequently truncated from the original size of 93 nucleotides with a dissociation constant (K D) of 3.6 × 10−7 M to just 11 nucleotides, with this truncation resulting in a two orders of magnitude improvement of the K D to 1.7 × 10−9 M [43]. This 11-nucleotide aptamer (11-mer), the shortest aptamer reported to date, was subsequently exploited in FRET-based assay, achieving picomolar detection limits, with the only required end-user intervention being sample addition and quantitative detection achieved in just one minute [44].
Here, we detail the selection and characterisation of a second aptamer selected against β-conglutin, which we demonstrate binds to a different aptatope. The aptamer was selected using SELEX based on magnetic bead separation, and aptamer candidates were subsequently analysed using ion torrent sequencing. The affinity of the selected β-CBA II was determined in three independent laboratories using the different methodologies of surface plasmon resonance, radiolabelling filter-binding assay and microscale thermophoresis. Microscale thermophoresis was also used to explore if the β-CBA I and β-CBA II aptamers had different binding sites on the β-conglutin cognate target.
Materials and methods
Materials
Phosphate-buffered saline (10 mM phosphate, 138 mM NaCl, 2.7 mM KCl, pH 7.4), 1-ethyl-3-(dimethylaminopropyl) carboiimide (EDC), N-hydroxysuccinimide (NHS) and all other reagents were purchased from Sigma (Barcelona, Spain). Sodium chloride, sodium hydroxide 2 M and hydrochloric acid 6 M were purchased from Scharlau Chemie S.A. (Barcelona, Spain). SiGMAG-Carboxyl beads were obtained from Chemicell (Zaragoza, Spain) and the Tfi DNA Polymerase and 10-bp DNA ladder from Invitrogen (Spain). Certified TM Low Range Ultra Agarose and Precision Plus Protein TM standards were purchased from Bio-Rad (Barcelona, Spain). Oligonucleotides (HPLC purified and provided lyophilized) were purchased from BIOMERS (Ulm, Germany). All solutions were prepared in high-purity water obtained from the Milli-Q RG system (Barcelona, Spain).
Aptamer selection
Proteins from Lupinus albus seeds were extracted, purified and characterised, obtaining a pure isolate of β-conglutin. β-Conglutin was linked to SiGMAG-Carboxyl magnetic beads via carbodiimide coupling, and the bioconjugation was confirmed using peptide mass fingerprinting (PMF) as previously described [41].
The DNA library used consisted of a 94-mer DNA sequence containing a random region of 50 nucleotides flanked by primer binding regions: 5′-agc tcc aga aga taa att aca gg- n(50)-caa cta gga tac tat gac ccc-3′.
In the initial round of selection, the DNA library pool (300 pmol) was denatured at 95 °C for 5 min and immediately cooled to 4 °C, after which 5 μl of magnetic beads functionalised with β-conglutin was added and the volume was brought to a total volume of 100 μl with binding buffer (10 mM phosphate, 138 mM NaCl, 2.7 mM KCl, 1.5 mM MgCl2, pH 7.4), and the mixture incubated for 30 min at 18 °C using tilt rotation. Following incubation, the beads were washed several times with 500 μl of binding buffer. After the first round, a negative selection step was introduced prior to the positive selection step, in order to remove non-specific binders. Selected DNA was incubated with 5 μl of control beads (magnetic beads with no protein attached and ethanolamine blocked), and as before, the volume was brought to 100 μl with the binding buffer for incubation at 18 °C for 30 min, and the unbound DNA was subsequently used for the positive selection step. The obtained library pool was amplified by PCR, and single-stranded DNA (ssDNA) was generated by a combination of asymmetric PCR and exonuclease digestion using PTO forward primer and addition of T7 Gene 6 exonuclease as previously described [45]. Highly purified ssDNA was obtained using the QUIAX II kit, and the ssDNA obtained from each round was used as the initial ssDNA pool for the following round of SELEX.
Evolution was followed after each round of SELEX using pilot PCR of nucleic acids that bound to the matrix (negative selection) and nucleic acids that bound specifically to β-conglutin (positive selection). A small aliquot of the SELEX pool was amplified for 4–14 PCR cycles, in order to elucidate the optimal number of amplification cycles. Following PCR, the amplified products from the negative and positive selection were analysed using agarose gel electrophoresis, with an indication of successful evolution being inferred by an increasing width of the band for positive selection and a decreasing band for negative selection. After an indication of success had been observed, the SELEX pool from the seventh round was sequenced using ion torrent sequencing and analysed using Galaxy software to identify any consensus motifs.
Binding affinity studies
Surface plasmon resonance (SPR) was performed using the BIAcore 300 (Biacore Inc.). Proteins of interest (β-conglutin, α-conglutin, γ-conglutin) were immobilised on separate channels of a CM5 sensor chip activated with EDC/NHS (30 μl of a 1:1 mixture of EDC (400 mM) and NHS (100 mM)), followed by injection of 200 μg/ml protein at a flow rate of 5 μl/min. Following immobilisation of the proteins, any unreacted NHS esters were deactivated via injection of an excess of ethanolamine hydrochloride (1 M). Unbound proteins were then washed from the surface using 2 M NaCl and 10 mM NaOH. The aptamer candidates were diluted in binding buffer to a final concentration of 1 μM and injected for 6 min at a flow rate of 5 μl/min followed by a 3-min stabilisation and 10-min dissociation. The binding was analysed through the corresponding changes in the refractive index of the optical signal, and expressed as resonance units (RU).
Microscale thermophoresis (MST) binding experiments were carried out with 5 nM of β-CBA II aptamer labelled with a Cy5 fluorophore in the 5′ end in binding buffer (10 mM phosphate, 138 mM NaCl, 2.7 mM KCl, 1.5 mM MgCl2, 0.1 % Tween, pH 7.4) with a range of concentrations of β-conglutin (0.056–1845 nM) at 20 % MST power and 20 % LED power in standard capillaries on a Monolith NT.115 pico device at 25 °C (NanoTemper Technologies, Munich, Germany). A ligand-dependent quenching effect was detected, and the raw fluorescence was thus used for analysis. The recorded fluorescence was normalised to fraction bound (0 = unbound, 1 = bound), processed using the KaleidaGraph 4.5 software and fitted using the K D fit formula derived from the law of mass action.
For the radiolabelling filter-binding assay, 1 μl (0.5 nM) of aptamer labelled with radioactive phosphate in the 5′ end, in binding buffer (10 mM phosphate, 138 mM NaCl, 2.7 mM KCl, 1.5 mM MgCl2, 1 mg/ml BSA, pH 7.4), was incubated with a range concentrations of β-conglutin (0.001–1000 nM) at room temperature (RT) for 30 min. The nitrocellulose filter was incubated with 0.3 M KOH for 15 min at RT followed by incubation with washing buffer (10 mM phosphate, 138 mM NaCl, 2.7 mM KCl, 1.5 mM MgCl2, pH 7.4) for a further 15 min. Finally, the radiolabelled aptamer-β-conglutin sample was applied to the filter, washed and transferred to the exposure cassette, and the phosphor screen was placed on the top of the filter for 2 h. Imaging was achieved using a Phosphorimager (Fuji FLA3000 phosphorimager), and the data was processed using AIDA and GraphPad Prism programs.
Duplicates were performed for each binding assay.
Truncation studies
Two truncation approaches were pursued; in the first, the primer region at either the 3′ terminus or 5′ terminus was removed, whilst in the second approach, various different truncated sequences postulated to be loops via the simulated 2D structure were generated using m-fold [43].
Competitive assay to demonstrate duality of β-CBA I and β-CBA II aptamers
In order to probe whether the β-CBA I and β-CBA II aptamers had the same or different binding sites, a competitive assay was carried out using MST, and following confirmation of duality, SPR was carried out to monitor sandwich aptacomplex formation.
In MST analysis, 500 nM β-conglutin was pre-incubated in binding buffer (10 mM phosphate, 138 mM NaCl, 2.7 mM KCl, 1.5 mM MgCl2, 0.1 % Tween, pH 7.4) with 5 nM of Cy5-β-CBA II. This complex was then incubated with increasing amounts of 11-mer aptamer (0.061–500 nM). As controls, Cy5-β-CBA II alone and β-conglutin/Cy5-β-CBA II were included in the experiments. The analysis was performed at 20 % MST power and 20 % LED power in standard capillaries on a Monolith NT.115 pico device at 25 °C (NanoTemper Technologies, Munich, Germany). Two types of data analyses were performed. Due to the previously detected β-conglutin-dependent quenching effect on the Cy5-β-CBA II aptamer, the raw fluorescence was checked for changes in the fluorescence upon addition of increasing amounts of 11-mer aptamer. In a second analysis, thermophoresis and temperature jump was used to detect changes in the thermophoretic movement of the Cy5-β-CBA II-β-conglutin complex upon addition of increasing amounts of 11-mer aptamer and the recorded fluorescence was normalised to fraction bound (0 = unbound, 1 = bound), processed using the software KaleidaGraph 4.5 and fitted using the K D fit formula derived from the law of mass action. Duplicate measurements were performed for each experimental setup.
To confirm these studies, SPR was performed with BIAcore 3000. Streptavidin was immobilised on separate channels of a CM 5 sensor chip activated with EDC/NHS (30 μl of a 1:1 mixture of EDC (400 mM) and NHS (100 mM)) followed by injection of 200 μg/ml streptavidin at a flow rate of 5 μl/min. Following immobilisation of streptavidin, unreacted NHS esters were deactivated via injection of an excess of ethanolamine hydrochloride (1 M). Biotinylated aptamer (10 μM) was injected into one channel. Unbound aptamer or streptavidin (control) was then removed from the surface via washing with 2 M NaCl and 10 mM NaOH. β-Conglutin was diluted in binding buffer (10 mM phosphate, 138 mM NaCl, 2.7 mM KCl, 1.5 mM MgCl2, pH 7.4) to a final concentration of 100 nM and injected for 6 min at a flow rate of 10 μl/min followed by a 3-min stabilisation and 2-min dissociation. Subsequently, 1 μM of the reporter aptamer diluted in binding buffer was injected for 6 min at a flow rate of 5 μl/min, again followed by a 3-min stabilisation and 10-min dissociation. The binding was analysed through the corresponding changes in the refractive index of the optical signal, and expressed as resonance units (RU). The chip was regenerated by 2 M NaCl and 10 mM NaOH. A channel without immobilised capture aptamer was used as a control.
Results and discussion
Selection of β-conglutin binding aptamer II
Selection of β-conglutin binding aptamer II (β-CBA II) was performed using magnetic partitioning. Following the second round of SELEX, prior to incubation with the protein-functionalised magnetic beads, a negative selection step was included, where the amplified DNA, followed by ssDNA generation, was initially incubated with naked magnetic beads (i.e. beads blocked with ethanolamine but without target). This negative selection was subsequently included in every round of SELEX to remove the non-specific sequences that bind to the beads rather than the target, thus increasing the selectivity of ssDNA to β-conglutin. Evolution was monitored after each round of SELEX by carrying out pilot PCR with ssDNA eluted from both negative and positive selection. After six rounds of SELEX, the vast majority of the ssDNA was binding to the target, where not only was there an increasing difference in the amount of DNA obtained from each round of negative and positive selection, but additionally, a decreasing number of PCR cycles were required to amplify the DNA (see Electronic Supplementary Material (ESM), Fig. S1).
Gel electrophoresis analysis of each of the negative and positive rounds of selection gave a clear indication that there was evolution of the pool towards the target, and ion torrent sequencing from the enriched DNA pool of the seventh round was carried out and consensus motifs identified. As can be seen in Table 1, this analysis identified just five major aptamer candidates, implying a successful evolution (Table 1).
Binding affinity studies
These five aptamer candidates for β-conglutin were evaluated in a screening manner using SPR with a BIACORE instrument. β-Conglutin (positive) and α- and γ-conglutins (negative) were immobilised on a CM5 chip, with another control being a blocked, non-functionalised surface. The five aptamer candidates were then flowed over these surfaces, and sequence 3 was observed to have significant affinity for β-conglutin whilst having no interaction with the other globulin subunits, demonstrating the specificity of this aptamer candidate (Fig. 1). The dissociation constant (K D) of sequence 3, now termed β-CBA II, was estimated using the Langmuir model, via the analysis of the binding with a range of concentrations (62–2000 nM) of β-conglutin. The resulting K D was 24 nM, and a good fit for the model was obtained as demonstrated by the chi2 value of 1.84 (Fig. 2a).
In order to corroborate the results obtained, evaluation of the affinity of the selected aptamer was performed in two independent laboratories (2Bind GmBH (www.2bind.de) and Mayer’s Lab (www.mayerlab.de)), using the different methodologies of microscale thermophoresis and filter radiolabelling, with MST allowing determination of the K D in free solution, whilst radiolabelling exploits immobilisation of the β-conglutin, with both using labelled aptamer.
MST was carried out with Cy5-labelled β-CBA II in constant range (5 nM) and wide range of β-conglutin (0.056–1845 nM). A ligand-dependent quenching effect was detected, showing decreasing fluorescence with a concomitant increase in aptamer concentration. This quenching was corroborated using denatured and native β-conglutin, where no difference in fluorescence was observed in denatured samples (high and low concentrations of β-conglutin), whilst a significant difference in fluorescence was observed with native β-conglutin (ESM Fig. S2). Consequently, raw fluorescence was used for the analysis. MST confirmed that the β-CBA II aptamer binds with high affinity and specificity to β-conglutin with a K D of 12 nM (Fig. 2b).
Radiolabelling filter-binding assay uses a filter of nitrocellulose paper, which is negatively charged and immobilises proteins via electrostatic interaction. DNA is inherently negatively charged due to the phosphate backbone and will thus be repelled by the filter, and only high-affinity aptamer that binds to β-conglutin will be present on the filter following interaction and washing. Subsequently, the presence of bound aptamer will be detected via the radioactive phosphate found in the 5′ terminus of the aptamer. A constant amount of aptamer is mixed with a range of concentrations of β-conglutin (0.001–1000 nM). A non-specific library was used as one negative control, with the other negative control being bare filter exposed to either aptamer or non-specific library. The high-affinity binding of the β-CBA II aptamer to β-conglutin was again confirmed and a K D of 1.81 nM obtained (Fig. 2c).
Truncation studies
SPR was used to evaluate the affinity of the various truncated sequences, which were flowed over to immobilised β-conglutin on the surface of a CM5 chip. The first approach for the truncation of β-CBA II evaluated was to remove the primer sequences at either the 3′ terminus (1), the 5′ terminus (2) or both termini (3) (Table 2a). In another approach, the β-CBA II aptamer was postulated using the m-fold program (ESM Fig. S5) and all the loops predicted in the 2D predicted structure of the aptamer were selected as truncated sequences (Table 2b). As can be seen in Fig. 3, the sequences present at the 5′ terminus (2) play a role in the binding as a lower K D was obtained (K D = 2.6 × 10−7 M), whilst the sequences present in the 3′ terminus are observed to be essential for the binding (1) and the studied truncated sequences were not observed to improve binding.
SPR experiments showing the interaction between truncated aptamer sequences obtained after removal of primer regions (a) or based on the analysis of simulated structures (b) to β-conglutin protein immobilised on the surface of the CM5 Biacore chip and DNA control: thrombin-binding aptamer (TBA). The binding of sequences is represented in resonance units (RU) (i) and normalised by the molecular weight of each sequence (ii)
Competition assay to determine duality of β-CBA I and β-CBA II aptamers
The aim of the competitive assay was to determine if the two β-conglutin binding aptamers (11-mer truncated β-CBA I and β-CBA II) bind to the same or different aptatopes on the protein. The strategy of the competition assay was to keep a saturated complex of β-conglutin (500 nM) and Cy5-β-CBA II (5 nM) constant, whilst titrating the 11-mer aptamer. Two types of analyses were performed: firstly, fluorescent measurement as the Cy5-β-CBA II showed a ligand-dependent quenching effect as previously described and, secondly, thermophoresis incorporating a temperature jump.
The analysis in the fluorescence showed that the quenching effect is still present, indicating that β-CBA II remains bound at all concentrations of the 11-mer β-CBA I (ESM Fig. S3). The thermophoresis analysis incorporating a temperature jump showed a binding event with a K D of 4.3 × 10−9 M (Fig. 4a), in agreement with the previously reported K D of 1.3 nM for the 11-mer. The competition assay clearly indicates that both aptamers bind to different binding sites on the surface of β-conglutin.
Dual-aptamer-based sandwich assay. a Competitive binding assay by MST, using constant complex (β-conglutin-β-CBA II aptamer-Cy5) and adding different concentrations of 11-mer aptamer. b Sandwich assay by SPR: the binding assays were performed using 10 μM biotin-β-CBA II aptamer as capture aptamer immobilised on streptavidin CM5 chip, 100 nM β-conglutin and 1 μM reporter aptamer (β-CBA II, β-CBA I, 11-mer or scrambled 11-mer)
In order to confirm that both aptamers could simultaneously bind to the β-conglutin, a sandwich assay using β-CBA II as capture aptamer, followed by the addition of 100 nM β-conglutin and 1 μM reporter aptamer (β-CBA I, β-CBA I-11-mer, β-CBA II or scrambled 11-mer), was detected by SPR. The SPR analysis showed that both aptamers could bind simultaneously to β-conglutin as there is an increase in the sensorgram when full-length β-CBA I or 11-mer is added comparing with scrambled 11-mer where no significant change is achieved or the same aptamer β-CBA II where a decrease is observed as both aptamers, capture and reporter, are competing for the β-conglutin (Fig. 4b). Additional analysis was carried out to ensure that there was no binding between the two aptamers in the absence of β-conglutin (ESM Fig. S4).
Conclusions
In conclusion, we report on the selection of a second aptamer, β-CBA II, against the anaphylactic allergen β-conglutin with high affinity and specificity. Microscale thermophoresis technology and radiolabelling filter-binding assay confirmed the affinity and specificity of β-CBA II. Competition assays monitored using microscale thermophoresis (MST) demonstrated that the previously selected and truncated β-CBA I and the newly selected β-CBA II bind to different aptatopes on the protein target. Finally, SPR was used to demonstrate the formation of sandwich aptacomplexes of immobilised β-CBA II with β-conglutin and either full-length β-CBA I or truncated 11-mer. Future work will focus on exploiting this sandwich aptacomplex in biosensor and apta-amplification approaches.
References
Adams GP, Schier R (1999) Generating improved single-chain Fv molecules for tumor targeting. J Immunol Methods 231(1–2):249–260
Neri D, Momo M, Prospero T, Winter G (1995) High-affinity antigen binding by chelating recombinant antibodies (CRAbs). J Mol Biol 246(3):367–373
Viti F, Tarli L, Giovannoni L, Zardi L, Neri D (1999) Increased binding affinity and valence of recombinant antibody fragments lead to improved targeting of tumoral angiogenesis. Cancer Res 59(2):347–352
Han K, Liang Z, Zhou N (2010) Design strategies for aptamer-based biosensors. Sens (Basel, Switzerland) 10(5):4541–4557
Ellington AD, Szostak JW (1990) In vitro selection of RNA molecules that bind specific ligands. Nature 346(6287):818–822
Tuerk C, Gold L (1990) Systematic evolution of ligands by exponential enrichment: RNA ligands to bacteriophage T4 DNA polymerase. Sci (New York, NY) 249(4968):505–510
Tasset DM, Kubik MF, Steiner W (1997) Oligonucleotide inhibitors of human thrombin that bind distinct epitopes. J Mol Biol 272(5):688–698
Bai Y, Feng F, Zhao L, Wang C, Wang H, Tian M, Qin J, Duan Y, He X (2013) Aptamer/thrombin/aptamer-AuNPs sandwich enhanced surface plasmon resonance sensor for the detection of subnanomolar thrombin. Biosens Bioelectron 47:265–270
Daniel C, Melaine F, Roupioz Y, Livache T, Buhot A (2013) Real time monitoring of thrombin interactions with its aptamers: insights into the sandwich complex formation. Biosens Bioelectron 40(1):186–192
Park JH, Cho YS, Kang S, Lee EJ, Lee GH, Hah SS (2014) A colorimetric sandwich-type assay for sensitive thrombin detection based on enzyme-linked aptamer assay. Anal Biochem 462:10–12
Pinto A, Bermudo Redondo MC, Ozalp VC, O'Sullivan CK (2009) Real-time apta-PCR for 20 000-fold improvement in detection limit. Mol BioSyst 5(5):548–553
Romhildt L, Pahlke C, Zorgiebel F, Braun HG, Opitz J, Baraban L, Cuniberti G (2013) Patterned biochemical functionalization improves aptamer-based detection of unlabeled thrombin in a sandwich assay. ACS Appl Mater Interfaces 5(22):12029–12035
Sosic A, Meneghello A, Antognoli A, Cretaio E, Gatto B (2013) Development of a multiplex sandwich aptamer microarray for the detection of VEGF165 and thrombin. Sens (Basel, Switzerland) 13(10):13425–13438
Sosic A, Meneghello A, Cretaio E, Gatto B (2011) Human thrombin detection through a sandwich aptamer microarray: interaction analysis in solution and in solid phase. Sens (Basel, Switzerland) 11(10):9426–9441
Lee J-e, Kim J, Lee S, Kim J, Mah S, Gu M (2013) In-situ on-fabric one-touch colorimetric detection using aptamer-conjugated gold nanoparticles. BioChip J 7(2):180–187
Liu J, Yang X, Wang K, Wang Q, Liu W, Wang D (2013) Solid-phase single molecule biosensing using dual-color colocalization of fluorescent quantum dot nanoprobes. Nanoscale 5(22):11257–11264
Vinkenborg JL, Karnowski N, Famulok M (2011) Aptamers for allosteric regulation. Nat Chem Biol 7(8):519–527
Wang Q, Zhou C, Yang X, Liu L, Wang K (2014) Probing interactions between human lung adenocarcinoma A549 cell and its aptamers at single-molecule resolution. J Mol Recognit JMR 27(11):676–682
Min K, Jo H, Song K, Cho M, Chun Y-S, Jon S, Kim WJ, Ban C (2011) Dual-aptamer-based delivery vehicle of doxorubicin to both PSMA (+) and PSMA (−) prostate cancers. Biomaterials 32(8):2124–2132
Min K, Song KM, Cho M, Chun YS, Shim YB, Ku JK, Ban C (2010) Simultaneous electrochemical detection of both PSMA (+) and PSMA (−) prostate cancer cells using an RNA/peptide dual-aptamer probe. Chem Commun (Camb) 46(30):5566–5568
Jo H, Youn H, Lee S, Ban C (2014) Ultra-effective photothermal therapy for prostate cancer cells using dual aptamer-modified gold nanostars. J Mater Chem B 2(30):4862–4867
Jo H, Her J, Ban C (2015) Dual aptamer-functionalized silica nanoparticles for the highly sensitive detection of breast cancer. Biosens Bioelectron 71:129–136
Fang LX, Huang KJ, Liu Y (2015) Novel electrochemical dual-aptamer-based sandwich biosensor using molybdenum disulfide/carbon aerogel composites and Au nanoparticles for signal amplification. Biosens Bioelectron 71:171–178
Ruslinda AR, Penmatsa V, Ishii Y, Tajima S, Kawarada H (2012) Highly sensitive detection of platelet-derived growth factor on a functionalized diamond surface using aptamer sandwich design. Analyst 137(7):1692–1697
Abbaspour A, Norouz-Sarvestani F, Noori A, Soltani N (2015) Aptamer-conjugated silver nanoparticles for electrochemical dual-aptamer-based sandwich detection of Staphylococcus aureus. Biosens Bioelectron 68:149–155
Hu PP, Liu H, Zhan L, Zheng LL, Huang CZ (2015) Coomassie brilliant blue R-250 as a new surface-enhanced Raman scattering probe for prion protein through a dual-aptamer mechanism. Talanta 139:35–39
Ahmad Raston NH, Gu MB (2015) Highly amplified detection of visceral adipose tissue-derived serpin (vaspin) using a cognate aptamer duo. Biosens Bioelectron 70:261–267
Rinker S, Ke Y, Liu Y, Chhabra R, Yan H (2008) Self-assembled DNA nanostructures for distance-dependent multivalent ligand-protein binding. Nat Nano 3(7):418–422
Zhao J, Zhang Y, Li H, Wen Y, Fan X, Lin F, Tan L, Yao S (2011) Ultrasensitive electrochemical aptasensor for thrombin based on the amplification of aptamer–AuNPs–HRP conjugates. Biosens Bioelectron 26(5):2297–2303
Ogawa A, Samoto M, Takahashi K (2000) Soybean allergens and hypoallergenic soybean products. J Nutr Sci Vitaminol 46(6):271–279
Sanchez-Monge R, Lopez-Torrejon G, Pascual CY, Varela J, Martin-Esteban M, Salcedo G (2004) Vicilin and convicilin are potential major allergens from pea. Clin Exp Allergy J Br Soc Allergy Clin Immunol 34(11):1747–1753
Scurlock AM, Burks AW (2004) Peanut allergenicity. Ann Allergy Asthma Immunol Off Publ Am Coll Allergy Asthma Immunol 93(5 Suppl 3):S12–S18
Teuber SS, Sathe SK, Peterson WR, Roux KH (2002) Characterization of the soluble allergenic proteins of cashew nut (Anacardium occidentale L.). J Agric Food Chem 50(22):6543–6549
Shewry PR, Napier JA, Tatham AS (1995) Seed storage proteins: structures and biosynthesis. Plant Cell 7(7):945–956
Holden L, Sletten GB, Lindvik H, Faeste CK, Dooper MM (2008) Characterization of IgE binding to lupin, peanut and almond with sera from lupin-allergic patients. Int Arch Allergy Immunol 146(4):267–276
Magni C, Herndl A, Sironi E, Scarafoni A, Ballabio C, Restani P, Bernardini R, Novembre E, Vierucci A, Duranti M (2005) One- and two-dimensional electrophoretic identification of IgE-binding polypeptides of Lupinus albus and other legume seeds. J Agric Food Chem 53(11):4567–4571
Campbell CP, Yates DH (2010) Lupin allergy: a hidden killer at home, a menace at work; occupational disease due to lupin allergy. Clin Exp Allergy J Br Soc Allergy Clin Immunol 40(10):1467–1472
Koplin JJ, Martin PE, Allen KJ (2011) An update on epidemiology of anaphylaxis in children and adults. Curr Opin Allergy Clin Immunol 11(5):492–496
Sanz ML, de Las Marinas MD, Fernandez J, Gamboa PM (2010) Lupin allergy: a hidden killer in the home. Clin Exp Allergy J Br Soc Allergy Clin Immunol 40(10):1461–1466
Goggin DE, Mir G, Smith WB, Stuckey M, Smith PM (2008) Proteomic analysis of lupin seed proteins to identify conglutin Beta as an allergen, Lup an 1. J Agric Food Chem 56(15):6370–6377
Nadal P, Pinto A, Svobodova M, Canela N, O'Sullivan CK (2012) DNA aptamers against the Lup an 1 food allergen. PLoS One 7(4):e35253
Svobodova M, Mairal T, Nadal P, Bermudo MC, O'Sullivan CK (2014) Ultrasensitive aptamer based detection of beta-conglutin food allergen. Food Chem 165:419–423
Nadal P, Svobodova M, Mairal T, O'Sullivan CK (2013) Probing high-affinity 11-mer DNA aptamer against Lup an 1 (beta-conglutin). Anal Bioanal Chem 405(29):9343–9349
Mairal T, Nadal P, Svobodova M, O'Sullivan CK (2014) FRET-based dimeric aptamer probe for selective and sensitive Lup an 1 allergen detection. Biosens Bioelectron 54:207–210
Svobodova M, Pinto A, Nadal P, OS CK (2012) Comparison of different methods for generation of single-stranded DNA for SELEX processes. Anal Bioanal Chem 404(3):835–842
Acknowledgments
This work was supported by funding from the national project RecerCaixa (CO074670 APTALUP).
Author information
Authors and Affiliations
Corresponding author
Ethics declarations
Conflict of interest
The authors declare that they have no competing interests.
Electronic supplementary material
Below is the link to the electronic supplementary material.
ESM 1
(PDF 1971 kb)
Rights and permissions
About this article
Cite this article
Jauset Rubio, M., Svobodová, M., Mairal, T. et al. β-Conglutin dual aptamers binding distinct aptatopes. Anal Bioanal Chem 408, 875–884 (2016). https://doi.org/10.1007/s00216-015-9179-z
Received:
Revised:
Accepted:
Published:
Issue Date:
DOI: https://doi.org/10.1007/s00216-015-9179-z