Abstract
Quantum chemical calculations based on density functional theory were used to analyze the potentially possible reactions between oxotitanium(IV) tetraphenylporphyrin ((TPP)Ti = O) and benzoyl peroxide (BP). Wavefunction analyses were performed, and electrostatic potential maps were calculated for (TPP)Ti = O and BP molecules. It was shown that the oxygen extra ligand is the most reactive part in (TPP)Ti = O. The structures of the possible inner- and outer-sphere complexes of (TPP)Ti = O with BP and their IR spectra were calculated, and the energies of their formation were estimated. The formation of an outer-sphere complex is the most thermodynamically probable process in the reaction of (TPP)Ti = O and BP. This interaction does not alter the state of the peroxy bond in the initiator molecule. A scheme was suggested to explain the reasons for the accelerating effect of oxotitanium(IV) porphyrins on the radical polymerization of methyl methacrylate reported in the literature. This scheme presumes that the polymerizing system contains a metal-centered radical, a product of the growing radical addition to the metalloporphyrin. This particle can form an inner-sphere complex with BP, which then undergoes homolytic decomposition.
Similar content being viewed by others
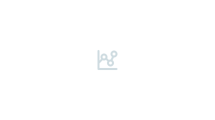
Avoid common mistakes on your manuscript.
1 Introduction
The potential possibility to use metalloporphyrins as initiators of controlled synthesis of macromolecules was discovered rather a long time ago [1]. However, many aspects of the mechanism of their action in polymerization remain unclear so far. This is due to the fact that metalloporphyrins (especially those containing transition metal atoms) exhibit catalytic activity in many reactions and can react with various components of the reaction mixture during polymerization. The same corresponds to the products of these reactions. The diversity of reactions that occur with the involvement of metalloporphyrins in syntheses of macromolecules makes it almost impossible to study the mechanism of such polymerization processes experimentally. In such situations, quantum chemical simulation is perhaps the only way to obtain information about the process elementary acts.
The range of nontransition metal porphyrins studied for their activity as regulators of radical polymerization is rather broad. The situation is completely different in the case of transition metal porphyrins. The action mechanism of cobalt and rhodium porphyrin that act as agents in catalytic chain transfer and catalytic inhibition has been studied rather well [2, 3]. Only a few experimental works on the effect of titanium porphyrins 1–3 (Scheme 1) on the polymerization process are reported in the literature [4, 5].
According to data available in the literature, oxotitanium(IV) porphyrins 1 and 2 (hereinafter, an oxotitanium(IV) porphyrin is generally designated as PTi = O) can be used as agents for controlled synthesis of macromolecules since they affect all stages of the polymerization process (chain initiation, growth and termination). In this case, the mechanism of this process has not been clarified completely. Regarding the effect of PTi = O on the initiation stage, it was reported [5] that the effective activation energy of radical polymerization of methyl methacrylate initiated by benzoyl peroxide (BP) was reduced significantly upon addition of metalloporphyrins 1 and 2 (from 80 kJ/mol in case of initiation by BP only to 38 and 30 kJ/mol in case of initiation by BP in the presence of metal porphyrins 1 and 2, respectively). In view of this, it was assumed [5] that PTi = O forms an initiating redox system with benzoyl peroxide. According to the authors [5], initially PTi = O forms a charge-transfer complex with BP, which then decomposes to a π-cation radical ([PTi = O]+•), a benzoyloxy radical (PhCOO•) and a benzoate anion (PhCOO−). The authors [5] believe that complexation occurs due to coordination of the carbonyl group in BP to the titanium atom. This assumption was made on the basis of the fact that upon mixing solutions of metalloporphyrin 1 and BP, the characteristic absorbtion band of the carbonyl group (1759 cm−1) shifts by 11 cm−1. However, these data are obviously insufficient to characterize the mechanism of polymerization initiation.
Information about the mechanism of action of metalloporphyrins on polymerization can be very useful for the development of efficient methods of controlled polymer synthesis. The reactions occurring at the initiation stage in reactions of an initiator with some components of the polymerization mixture can affect significantly the entire subsequent pathway of the process and the structure and properties of the resulting polymer. Therefore, it seems important to gain knowledge about the possible reactions between the polymerization initiator and the metalloporphyrin under consideration. Theoretical studies on this issue are missing in the literature. Therefore, in this study I performed a quantum chemical analysis of the possible pathways of the coordination interaction of oxotitanium(IV) porphyrin with benzoyl peroxide (a polymerization initiator), estimated the energy characteristics of potential reactions and analyzed the structure of the intermediates. A scheme explaining the mechanism of the metalloporphyrin effect on the polymerization initiation stage was suggested.
2 Computational details
Quantum chemical calculations were performed by means of Priroda-06 software [6, 7] that uses Gauss-type basis sets to solve Kohn–Sham equations, as well as electron density decomposition in auxiliary basis to calculate Coulombic and exchange–correlation energy. The PBE density functional [8] and a three-exponent basis set consisting of compacted orbital sets of Gaussian type functions, viz. (5s1p)/[3s1p] for H, (11s6p2d)/[6s3p2d] for C and O and (17s13p8d)/[12s9p4d] for Ti, were used. The auxiliary basis sets are uncompressed sets of Gaussian type functions with (5s2p) size for H, (10s3p3d1f) size for C and O and (18s6p6d5f5g) size for Ti. The unrestricted formulation was used for open-shell systems. The calculations were performed for the gas phase. Optimization of the geometric parameters of the structures of interest was performed without symmetry restrictions. The ΔE values of the reactions were calculated for standard conditions. For some of the reactions, the ΔE values were also calculated taking the effect of the polar environment into account. In these cases, the solvent effect was taken into account in the framework of the polarized continuum model using the Gaussian’09 program [9] (single-point calculations of structures in methyl ethanoate optimized by the PBE/3z method described above were performed by the UB3LYP/6-31G(d,p) method). Oxotitanium(IV) tetraphenylporphyrin 3 ((TPP)Ti = O) was used in this work as the model oxotitanium(IV) porphyrin.
The adequacy of the quantum chemical method was demonstrated previously [10].
Wavefunction analysis and calculations of electrostatic potential (ESP) were performed using Multiwfn software [11]. The method of quantitative evaluation of ESP was described in the work [12]. The isosurface maps were rendered by VMD 1.9.3 program [13] based on the outputs of Multiwfn.
3 Results and discussion
3.1 Determination of reactive sites of the molecules
The reactivity of particles toward each other is usually considered within the framework of the transition state theory. However, the energy characteristics of reactions alone (activation barriers, thermal effects) may not be enough. To estimate the possibility of a reaction and its most favorable pathways, it is expedient to take into account the Coulombic interaction that depends on the polarity of the interacting particles. Information on frontier molecular orbitals may also be useful. Therefore, to determine the most reactive parts of molecules, the charge density distribution (i.e., the electrostatic potential map) should be studied along with their frontier molecular orbitals.
To determine the most reactive parts of interacting particles, the electrostatic potential of (TPP)Ti = O and BP molecules was calculated (the ESP maps are shown in Fig. 1; more detailed information on ESP is given in the ESI section). The global minimum of the (TPP)Ti = O molecule (− 49.0 kcal/mol) is located in the region of the oxygen extra ligand. The global maximum (23.2 kcal/mol) is located around the titanium atom, on the opposite side from the extra ligand. The global minima in the BP molecule (− 32.4 kcal/mol) are localized in the region of the O atoms of the carbonyl groups, while the maxima are localized in the region of the H atoms of the phenyl groups (19.3 kcal/mol). Based on these data, it can be believed that the oxygen extra ligand is the most reactive part of the (TPP)Ti = O molecule.
ESP-mapped molecular vdW surface of (TPP)Ti = O (a) and BP (b). The unit is in kcal/mol. Surface local minima and maxima of ESP are represented as blue and red spheres, respectively. The transparent ones correspond to the extrema at backside of graph. Cartesian coordinates and the values of all extrema are given in the ESI section
Previously [10], the frontier molecular orbitals of the (TPP)Ti = O molecule were calculated. It was shown that the orbitals localized on the titanium atom are rather high in energy. The frontier (most reactive) molecular orbitals are mainly localized on the C atoms of the porphyrin macrocycle and, to a small extent, on the extra ligand. Thus, based on the above, one should not expect that the titanium atom would actively participate in complexation with BP.
3.2 Simulation of complexation reactions
All the possible interaction variants were considered in the quantum chemical simulation of complexation reactions between BP and (TPP)Ti = O: (1) coordination of the oxygen atoms of the peroxide and carbonyl groups on the metal both on one side and on the opposite side from the extra ligand (inner-sphere complexes) and (2) interaction with the hydrogen atoms of the organic ligand of metalloporphyrin (outer-sphere complexes). A schematic drawing of the calculated structures, as well as the ΔE values of reactions of their formation, is shown in Scheme 2 (the general view of the complexes and Cartesian coordinates are provided in the ESI section).
3.2.1 Inner-sphere complexes
Structures 4–6 are inner-sphere complexes where the O–O bond is stretched considerably (approximately by 0.8 Å) compared to that in the free BP molecule, and its order is only 0.32 (for comparison, the O–O bond order in a free BP molecule is 0.84). Calculations revealed no complexes where the O atom of the peroxide group in BP is coordinated to the titanium atom on the same side as the extra ligand.
Complexes 4–6 contain two unpaired electrons with parallel spins, i.e., they have a triplet ground state. Spin density is distributed as follows: the first unpaired electron is mostly localized on the O atoms of the peroxide bond, while the second one is distributed over the meso-positions and N atoms of the porphyrin cycle. The spin density distribution in complex 6 is shown in Fig. 2. More detailed information on the spin density distribution in complexes 4–6 is shown in the ESI section.
As one can see from the calculated heat effects (ΔE values at Scheme 2), the formation of inner-sphere complexes is energetically unfavorable. It can be noted that, even with consideration for the effect of the polar solvent, the calculated ΔE for the formation of complex 6 amounts to 48.8 kJ/mol.
3.2.2 Outer-sphere complexes
Hydrogen bonds are formed upon interaction of O atoms belonging to BP with H atoms of metalloporphyrin phenyl groups. It is clear that in the case of this interaction, rather many variants of coordination interaction of BP with (TPP)Ti = O are possible. As an example, Scheme 2 shows three outer-sphere complexes of BP with (TPP)Ti = O (structures 7–9). As one can see from the calculated ΔE values, the formation of such complexes is energetically favorable. The formation of structure 8 is most likely from the energetics point of view. In addition to the bonds between the BP carbonyl groups and the H atoms of the phenyl group in the metalloporphyrin, it also contains a hydrogen bond between the oxygen extra ligand in the metalloporphyrin and the H atom of the phenyl group in BP. Complexes 7–9 are in singlet state and, unlike structures 4–6, no weakening of their peroxy bonds is observed.
Thus, from the energetics point of view, the formation of outer-sphere complexes is more likely. However, the state of the O–O bond in these structures does not change, and it is hardly possible to say that interaction of this kind would facilitate the decomposition of the initiator and result in the effects observed [5] at the polymerization initiation stage. Conversely, weakening of the O–O bond occurs in the case of inner-sphere complexes. However, the formation of these complexes is energetically unfavorable, judging by the calculated ΔE values.
3.3 IR spectra of the complexes
In order to clarify what kind of complexes corresponds to the IR spectra obtained earlier [5] in an experimental study on the complexation between the oxotitanium(IV) porphyrin complex and BP, the IR spectra of BP, (TPP)Ti = O and complexes 4–9 were calculated in this study. It was found that in complexes 7–9, the bands belonging to the vibrations of BP carbonyl groups shift by 6–11 cm−1 to lower frequencies, while the IR spectra of complexes 4–6 (corresponding to coordination of the O atoms of the BP molecule to the Ti atom) showed no signals around 1700 cm−1 at all (the calculated IR spectra are given in the ESI section). Thus, the assumption previously set forth in Ref. [5] was not confirmed. The data obtained in this study indicate that outer-sphere rather than inner-sphere interaction between (TPP)Ti = O and BP takes place to give structure 8. Its formation is most thermodynamically probable, while the signals of BP carbonyl groups in this complex are shifted by 11 cm−1 (as in experiment [5]).
3.4 What particle catalyzes the decomposition of benzoyl peroxide?
The results of the theoretical study presented above show that oxotitanium(IV) porphyrin alone does not catalyze the decomposition of benzoyl peroxide. Even if we assume that some of the inner-sphere complexes (4–6), in which the peroxide bond is weakened noticeably, are still formed (despite the large positive ΔE value), decomposition of such a complex by the heterolytic mechanism mentioned above [5] to give [PTi = O]+•, benzoyloxyl radical PhCOO• and benzoate anion is unlikely: the ΔE values calculated with consideration for the solvent effect are 73, 107 and 102 kJ/mol for structures 4, 5 and 6, respectively (if the solvent effect is not taken into account, the corresponding ΔE values are 333, 354 and 355 kJ/mol). In general, if we speak of the mechanism of further transformations of inner-sphere complexes, homolytic decomposition into [(PhCOO)(TPP)Ti = O]• and PhCOO• is much more likely: the calculated ΔE values for the latter reaction are 5, − 5 and − 4 kJ/mol for reactions involving structures 4, 5 and 6, respectively.
In the experimental study referred to above [5], the IR spectrum of a mixture of metalloporphyrin 1 and BP was recorded in CCl4. However, polymerization is carried out in the medium of the monomer (methyl methacrylate). Taking all the above into account, it can be assumed that the particle which actually catalyzes BP decomposition is formed at the very beginning of the polymerization process. Taking into account that the monomer concentration during polymerization is a few orders higher than the concentrations of the initiator and metalloporphyrin, it is logical to assume that this particle is a product of interaction of the metalloporphyrin with the methyl methacrylate radical (R•). Previously [10], the possible pathways of the reaction of (TPP)Ti = O with the methyl methacrylate radical were studied and it was shown that the metal-centered radical (TPP)Ti•–O–R was the most likely product. Since this intermediate can be formed at the very beginning of the polymerization process, it may be assumed that it is indeed the particle that accelerates BP decomposition. The results of calculations made in this study confirm this hypothesis. The complexation reaction of BP and (TPP)Ti•–O–R was simulated. It was found that as the titanium atom in the (TPP)Ti•–O–R intermediate and the carbonyl group in BP approach each other, complex 10 is formed. Its structure and the heat effect of its formation are shown in Fig. 3.
As shown in Fig. 3, the formation of this complex is energetically favorable. The peroxy bond in this complex is elongated considerably (its order is 0.28); hence, it can be believed that the formation of this complex is an intermediate stage of BP decomposition under the effect of the (TPP)Ti•–O–R intermediate. The spin density in the [(PhC(O)O)2∙∙∙(TPP)Ti–O–R]• complex is mainly localized on the oxygen atoms of the peroxy group (as shown in Fig. 2). More detailed information on the distribution of spin density in 10 is given in the ESI section.
The calculated energy change in the homolytic decomposition of complex 10 by the scheme
is − 7.3 kJ/mol. Thus, it can be concluded that the experimentally observed increase in the starting methyl methacrylate polymerization rate in the presence of (TPP)Ti = O initiated by BP may be due to the reactions presented in Scheme 3.
4 Conclusions
It has been shown by quantum chemical calculations that the most thermodynamically probable process is the formation of an outer-sphere complex between (TPP)Ti = O and BP. However, such a complexation does not result in weakening of the peroxy bond in the initiator molecule. Thus, the oxotitanium(IV) porphyrin alone does not form an initiating redox system with benzoyl peroxide. The accelerating effect of oxotitanium(IV) porphyrins on the radical polymerization described in the literature may be due to the presence of the metal-centered radical (TPP)Ti•–O–R, a product of addition of the methyl methacrylate growing radical to the metalloporphyrin, in the polymerization system. The (TPP)Ti•–O–R particle can catalyze BP decomposition by a homolytic mechanism, as indicated by the high thermodynamic probability of the following reactions:
References
Kadish KM, Smith KM, Guilard R (eds) (2000) The porphyrin handbook: applications: past, present and future, vol 6. Academic Press, New York
Wayland BB, Poszmik G, Mukerjee S (1994) Living radical polymerization of acrylates by organocobalt porphyrin complexes. J Am Chem Soc 116:7943–7944. https://doi.org/10.1021/ja00096a080
Heuts JPA, Forster DJ, Davis TP (2000) Mechanistic aspects of catalytic chain transfer polymerization. In: Boffa LS, Novak BM (eds) Transition metal catalysis in macromolecular design, Chapter 16. ACS Publications, Washington, pp 254–272. https://doi.org/10.1021/bk-2000-0760.ch016
Aida T, Inoue S (1996) Metalloporphyrins as initiators for living and immortal polymerizations. Acc Chem Res 29(1):39–48. https://doi.org/10.1021/ar950029l
Islamova RM, Nazarova SV, Koifman OI (2011) Porphyrins and their metal complexes in radical polymerization of vinyl monomers. Macroheterocycles 4(2):97–105. https://doi.org/10.6060/mhc2011.2.06
Laikov DN, Ustynyuk Y (2005) PRIRODA-04: a quantum-chemical program suite. New possibilities in the study of molecular systems with the application of parallel computing. Russ Chem Bull 54:820–826. https://doi.org/10.1007/s11172-005-0329-x
Laikov DN (1997) Fast evaluation of density functional exchange-correlation terms using the expansion of the electron density in auxiliary basis sets. Chem Phys Lett 281:151–156. https://doi.org/10.1016/S0009-2614(97)01206-2
Perdew JP, Burke K, Ernzerhof M (1996) Generalized gradient approximation made simple. Phys Rev Lett 77:3865–3868. https://doi.org/10.1103/PhysRevLett.77.3865
Frisch MJ, Trucks GW, Schlegel HB, Scuseria GE, Robb MA, Cheeseman JR, Scalmani G, Barone V, Mennucci B, Petersson GA, Nakatsuji H, Caricato M, Li X, Hratchian HP, Izmaylov AF, Bloino J, Zheng G, Sonnenberg JL, Hada M, Ehara M, Toyota K, Fukuda R, Hasegawa J, Ishida M, Nakajima T, Honda Y, Kitao O, Nakai H, Vreven T, Montgomery JA Jr, Peralta JE, Ogliaro F, Bearpark M, Heyd JJ, Brothers E, Kudin KN, Staroverov VN, Keith T, Kobayashi R, Normand J, Raghavachari K, Rendell A, Burant JC, Iyengar SS, Tomasi J, Cossi M, Rega N, Millam JM, Klene M, Knox JE, Cross JB, Bakken V, Adamo C, Jaramillo J, Gomperts R, Stratmann RE, Yazyev O, Austin AJ, Cammi R, Pomelli C, Ochterski JW, Martin RL, Morokuma K, Zakrzewski VG, Voth GA, Salvador P, Dannenberg JJ, Dapprich S, Daniels AD, Farkas O, Foresman JB, Ortiz JV, Cioslowski J, Fox DJ (2013) Gaussian 09, Revision D.01. Gaussian Inc, Wallingford CT
Friesen AK (2018) The reactions of oxotitanium(IV) tetraphenylporphyrin with methyl methacrylate radicals: a DFT study. Macroheterocycles 11(4):371–377. https://doi.org/10.6060/mhc181112f
Lu T, Chen F (2012) Multiwfn: a multifunctional wavefunction analyzer. J Comput Chem 33(5):580–592. https://doi.org/10.1002/jcc.22885
Lu T, Manzetti S (2014) Wavefunction and reactivity study of benzo[a]pyrene diol epoxide and its enantiomeric forms. Struct Chem 25(5):1521–1533. https://doi.org/10.1007/s11224-014-0430-6
Humphrey W, Dalke A, Schulten K (1996) VMD—visual molecular dynamics. J Mol Graph 14:33–38. https://doi.org/10.1016/0263-7855(96)00018-5
Acknowledgements
The calculations were performed using the equipment at the Center for collective use “Khimiya” (Chemistry) at Ufa Institute of chemistry UFRC RAS within the State task for 2017–2019 (AAAA-A17-117011910026-3).
Author information
Authors and Affiliations
Corresponding author
Additional information
Publisher's Note
Springer Nature remains neutral with regard to jurisdictional claims in published maps and institutional affiliations.
Electronic supplementary material
Below is the link to the electronic supplementary material.
Rights and permissions
About this article
Cite this article
Friesen, A.K. The complexation reaction of oxotitanium(IV) tetraphenylporphyrin with benzoyl peroxide: a DFT study. Theor Chem Acc 138, 54 (2019). https://doi.org/10.1007/s00214-019-2441-2
Received:
Accepted:
Published:
DOI: https://doi.org/10.1007/s00214-019-2441-2