Abstract
Parathyroid glands are endocrine organs which are located posterior to thyroid glands and control secretion of parathyroid hormone (PTH) in order to regulate blood calcium level. PTH maintains calcium homeostasis by acting on the bone, kidney, and small intestine. PTH deficiency leads to chronic hypocalcemia, organ calcinosis, kidney and heart failure, painful muscle spasms, neuromuscular problems, and memory problems. Since parathyroid cells have inadequate proliferation potential in culture conditions, their utilization as a cellular therapy option is very limited. Although studies conducted so far include parathyroid cell differentiation from various cell types, problems related to successful cellular differentiation and transplantation still remain. Recently, parathyroid tissue engineering has attracted attention as a potential treatment for the parathyroid-related diseases caused by hypoparathyroidism. Although major progression is made in the construction of tissue engineering protocols using parathyroid cells and biomaterials, PTH secretion to mimic its spontaneous harmony in the body is a challenge. This chapter comprehensively defines the derivation of parathyroid cells from various cell sources including pluripotent stem cells, molecular mechanisms, and tissue engineering applications.
Access provided by Autonomous University of Puebla. Download chapter PDF
Similar content being viewed by others
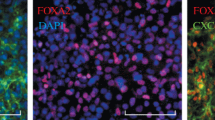
Keywords
1 Introduction
Parathyroid glands are small endocrine organs which are located at the posterior part of the thyroid gland. Parathyroid glands secrete parathyroid hormone (PTH) in response to low calcium levels to regulate calcium metabolism (Cipriani 2014). Two different cell types such as chief cells and oxyphil cells are present in the parathyroid glands (Taterra et al. 2019). Although the function of oxyphil cells is unknown, several investigations indicate that oxyphil cells contribute to PTH secretion in secondary hyperparathyroidism (Tanaka et al. 1996). Chief cells produce PTH in response to low calcium which triggers the calcium-sensitive receptor (CaSR) and initiates the G-protein messenger pathway. In contrast, the G-protein messenger pathway is blocked and PTH secretion is diminished when the blood calcium level is high which is related to the secretion of calcitonin from the thyroid gland (Brown and Limaiem 2020). Calcium is one of the most widely distributed inorganic elements which regulates bone development and maintenance in mammals by targeting kidney and intestine for reabsorption of calcium (Khan and Sharma 2018). Calcium homeostasis is driven by four important factors such as PTH, calcitonin, metabolic D3, and fibroblast growth factor 23 (FGF23) (Shaker and Deftos 2013).
PTH is the hormone which raises the blood calcium concentration by three main mechanisms:
-
PTH induces the generation of the active form of vitamin D, calcitriol, which plays role in the intestines to enhance calcium absorption.
-
PTH simplifies the transportation of calcium from bone into the blood by stimulating osteoclasts when the blood calcium concentration is decreased.
-
PTH increases the reabsorption of calcium directly in the kidney tubule. Therefore, the loss of calcium in the urine is decreased (Talmage and Mobley 2008).
Along with calcium homeostasis, PTH contributes to phosphate homeostasis. PTH inhibits phosphate reabsorption from the proximal tubule of the kidney, resulting in increased phosphate excretion through the urine. PTH, on the other hand, increases the absorption of phosphate from the stomach and bones into the blood. The final result of PTH release is a decrement in serum phosphate concentration (Jayakumar 2012). Parathyroid is a small endocrine organ that plays crucial roles in calcium and phosphate metabolism and regulates bone, kidney, and intestine tissue metabolism (Bro and Olgaard 1997). In the current chapter, we reviewed the differentiation of parathyroid cells from stem cells. We organized the current literature on development, molecular mechanism, differentiation, function, and tissue engineering of the parathyroid tissue.
2 PTH Mechanism and Biosynthesis
PTH is the main hormone which regulates calcium and phosphate homeostasis by acting on bone and kidney cells in the body (Renkema et al. 2008). PTH is a 115-amino acid precursor peptide that is extended at the amino (NH2)-terminus by a “pre” sequence that facilitates entry into the secretory system of cells. Propeptide PTH(1–84) is the main secreted and circulating form of the hormone, while the leader sequence is cleaved in the cell, giving rise to a straight peptide chain containing 84 amino acids, PTH(1–84). Ionized calcium, Ca+, in the extracellular fluid (ECF), appears to be the primary regulator of PTH generation and secretion.
Type 1 parathyroid hormone receptor (PTH1R) and type 2 parathyroid hormone receptor (PTH2R) which belong to the G-protein-coupled receptors family have been identified for PTH and parathyroid hormone-related protein (PTHrP) binding. PTHrP is secreted by a diverse range of cells throughout the life, including fetal and postnatal developmental stages. Surface epithelium of developing organs, mesenchyme, vascular smooth muscle, and the central nervous system are among the tissues that have been shown to release this hormone (Strewler 2009). PTH1R is activated by both PTH and PTHrP. Binding to this receptor triggers N-terminal PTH-like domain of the receptor which stimulates the cyclic AMP/Protein kinase A (PKA) pathway or calcium-dependent pathway (Mannstadt et al. 1999). PTH1R mRNA is mostly expressed in bone and renal tubules (Clemens et al. 2001). On the other hand, PTH2R has a very low affinity for PTHrP while it binds to PTH. Similar to PTH1R, it activates adenylyl cyclase and this causes an increase in intracellular cyclic AMP concentration (Mannstadt et al. 1999). PTH2R mRNA is expressed predominantly by adipocytes and keratinocytes in the skin (Sato et al. 2016).
By acting on the bone, kidney, and small intestine (Khan and Sharma 2018), PTH maintains calcium homeostasis. PTH exerts its effects on all bone cell types including osteocytes, osteoblasts, and osteoclasts. PTH first induces osteocyte osteolysis, resulting in the disintegration of the bone surface (Bellido et al. 2013). Ca is transported from the bone canalicular fluid into the osteocytes and then into the extracellular fluid as a result of this process. Then, PTH stimulates osteoclasts to promote bone resorption, releasing calcium and phosphate into the extracellular fluid. PTH exerts both anabolic and catabolic effects on bone tissue. PTH’s catabolic effect on bone is an increase in bone resorption (Silva et al. 2011) in response to hypocalcemic stimuli. Numerous in vivo and in vitro studies have shown that PTH indirectly increases bone resorption via osteoclast activation (Chambers et al. 1985; McSheehy and Chambers 1986). On the other hand, preclinical and clinical investigations have demonstrated PTH’s anabolic effect on bone tissue. In these studies, it was discovered that the use of PTH stimulates bone development (Neer et al. 2001; Greenspan et al. 2007; Iwaniec et al. 2007). The anabolic effect of PTH treatment is inversely proportional to the osteoblastic lineage in terms of bone mass (Yang et al. 2007). The hormone PTH, as previously stated, promotes and improves the growth of osteoblasts in primary calvarial cells as well as bone marrow-derived cells. Additionally, it has been demonstrated that intermittent PTH promotes the production of ossicles in immunodeficient animals following the implantation of bone marrow-derived cells on the dorsal surface of each mouse; mid-longitudinal skin incisions of approximately 1 cm in length were created (Pettway et al. 2005).
Physiological calcium reabsorption in the nephron occurs in the proximal convoluted tubule as well as the ascending Henle loop in the kidneys. By targeting the distal convoluted tubule and collecting duct, circulating PTH directly increases calcium reabsorption. In the proximal convoluted tubule, PTH inhibits phosphate reabsorption. Phosphate ions in serum form insoluble salts with calcium, lowering plasma calcium. As a result, phosphate ion reduction results in more ionized calcium in the blood (Khan and Sharma 2018). PTH increases the formation of 1-alpha-hydroxylase in the proximal convoluted tubule of the kidneys. This enzyme, 1-alpha-hydroxylase, is essential for the catalysis of the conversion of 25-hydroxycholecalciferol to active vitamin D-1,25-dihydroxycholecalciferol. Vitamin D is active and participates in calcium reabsorption in the distal convoluted tubule. Vitamin D facilitates calcium absorption in the small intestine via an active transcellular pathway and a passive paracellular mechanism. The transcellular route demands energy, but the paracellular route allows calcium to pass past tight junctions (Khan and Sharma 2018).
3 Development of Parathyroid Glands
The 4 parathyroid glands are divided into pairs – upper parathyroid glands and lower parathyroid glands – which are located in close proximity to thyroid glands in the adult body (Rosen and Bordoni 2020). The parathyroid glands are originated from the endoderm layer of the third and fourth pharyngeal pouches (Casale and Giwa 2019). The third pharyngeal pouch and the fourth pharyngeal pouch give rise to the lower parathyroid glands and the upper parathyroids respectively. The third pharyngeal pouch is split into dorsal and ventral parts. The dorsal part leads to the lower parathyroid gland, while the ventral part leads to the formation of the thymus. In the seventh week of gastrulation, the lower parathyroid glands separate from the posterior pharyngeal wall and follow the thymus tract to reach their final destination in the posterior thyroid (Rosen and Bordoni 2020). The fourth pharyngeal pouch is divided into dorsal and ventral parts. The dorsal part differentiates into the superior parathyroid gland and the ventral part differentiates into the ultimobranchial body. The parathyroid glands are separated from the pharyngeal wall and attached to the posterior surface of the thyroid when the seventh week of development is completed (Rosen and Bordoni 2020).
In the pharyngeal endoderm, 4 main transcription factors are responsible for the development of parathyroid precursor. These are Homeobox A3 (HOXA3), Paired box 1 (PAX1), EYA Transcriptional Coactivator And Phosphatase 1 (EYA1), and Paired box 9 (PAX9), all of which are involved in thymus development at the pharyngeal endoderm stage (Bingham et al. 2009). HOXA3 is expressed in both the third pouch endoderm and the neural crest mesenchyme, and its function has been demonstrated to be upstream of PAX1 and PAX9 (Su et al. 2001). PAX1 and PAX9 are members of the paired-box gene family, which is involved in the development of numerous organs (Neubueser et al. 1995). PAX9 deficiency results in an early failure of thymus, parathyroid, and ultimobranchial body development (Peters et al. 1998), whereas PAX1 deficiency results in hypoplastic parathyroid and thymus, as well as abnormal thymocyte maturation (Su et al. 2001). At E9.5–10.5, Eya1 is highly expressed in the pharyngeal arches, pouch endoderm, and surface ectoderm, including pharyngeal clefts. Following that, expression of Eya1 was detected in structures arising from the pharyngeal area, including the thymus and parathyroid. Without Eya1, the thymus and parathyroid fail to develop concurrently with the early activation of organogenesis (Xu et al. 2002).
Parathyroid glands are developed in the third pharyngeal pouches in mice at E11.5 (Cordier and Haumont 1980) whereas in both third and fourth pouches in humans starting at 5–6 weeks (Gilmour 1937; Liu et al. 2010). The cell fate decision starts in E11.5 for parathyroid and thymus. Primordium cells located at the third pharyngeal pouches differentiate into the parathyroid and the thymus in the presence of glial cell missing 2 (GCM2) and forkhead box N1 (FOXN1), respectively (Gordon et al. 2001). Additionally, FGF (Gardiner et al. 2012) and bone morphogenetic protein 4 (BMP4) (Gordon et al. 2010) signaling pathways regulate the differentiation of the parathyroid-thymus complex from the pharynx. FOXN1 expression is seen in the ventral/posterial prospective third pharyngeal pouch, also known as the anterior thymus mass, at E10.5 through E12.5, with BMP4 expression found in the surrounding mesenchyme (Bleul and Boehm 2005). A recent study has shown that FGF feedback antagonists from the sprouty gene family are important regulators of parathyroid organogenesis (Mason et al. 2006). During thymus/parathyroid organogenesis, sprouty proteins block FGF signaling at the cellular level. A decrease in the induction of GCM2 expression is caused by increased FGF signaling in the absence of sprouty proteins, which leads to the creation of a small GCM2+ domain by E11.5. GCM2 deficiency may be the cause of sprouty protein mutants’ hypoplasia of the parathyroid gland (Gardiner et al. 2012).
GCM is a transcription factor which was first identified in Drosophila which regulates the determination of neuronal or glial fate (Hosoya et al. 1995). GCM1 and GCM2 were characterized in mammalians as homologs of GCM in Drosophila (Kim et al. 1998). GCM2 is slightly expressed in second and third dorsal pouches at E9.5 and upregulated at E10.5 followed by limited expression in dorsal-anterior of the third pouch endoderm (Gordon et al. 2001). GCM2 is important for the survival and differentiation of parathyroid cells. When GCM2 is upregulated, PTH and CaSR which are downstream of parathyroid development pathways are activated. In the absence or reduced expression of the parathyroid GCM2 gene, as seen in GCM2 knockout mice (Günther et al. 2000) or cultured human parathyroid cells treated with GCM2 siRNA (Mizobuchi et al. 2009), CaSR expression is reduced too. CaSR is a marker for early parathyroid differentiation (Liu et al. 2007). GCM2 activates the CaSR gene by binding to GCM response elements in the CASR promoters P1 and P2, which are located in the CASR promoter (Canaff et al. 2008). Thus, GCM2 and CaSR are mechanistically linked to the evolutionarily related parathyroid glands (Okabe and Graham 2004).
Besides, C-C motif chemokine ligand 21 (CCL21) activates the parathyroid domain of the receptor which is expressed at E11.5, and it is responsible for appealing lymphoid progenitors into the thymus domain (Liu et al. 2006). The conservation of CaSR and CCL21 expression in the parathyroid domain is a GCM2-dependent process, whereas the expression of CaSR and CCL21 is GCM2-independent. In discrepancy, PTH initiation and maintenance is a GCM2-dependent process at E12 (Liu et al. 2007). Because PTH is the primary target of GCM2, PTH expression was never observed in GCM2 null mice and parathyroid cells apoptosis was detected in GCM2 null mice (Liu et al. 2007). As a result of the absence of parathyroid glands in GCM2 null mice, it is believed that GCM2 gene is required for parathyroid gland development and survival at the earliest stage following organ specification during embryogenesis (Günther et al. 2000). Additionally, it is predicted that GCM2 plays a critical role in parathyroid function during mineral homeostasis. A series of analyses indicated that GCM2, in conjunction with MAF BZIP transcription factor B (MAFB) and GATA binding protein 3 (GATA3), regulates serum calcium concentration via CaSR binding (Mizobuchi et al. 2009) and followed by promoting PTH secretion (Yamada et al. 2019).
4 Derivation of Parathyroid Cells from Pluripotent Stem Cells
Elimination of parathyroid glands during thyroid surgery or radiotherapy of neck cancers results in parathyroid-related diseases such as hypoparathyroidism and hypocalcemia (Marx 2000). The lack of parathyroid glands and the resulting disorders lead to heart and kidney failure, painful muscle spasms, and neuromuscular problems (Khan and Sharma 2018). Development of parathyroid tissue engineering strategies, cellular therapy solutions, and successful treatment strategies is required to reduce or completely eliminate the hypoparathyroidism-related problems.
Therefore, derivation of parathyroid cells with parathyroid hormone production capacity in a laboratory might be a cell therapy-based solution. The recent studies on patient-specific therapies using human induced pluripotent stem cells are potential candidates for treatment (Fig. 1).
The molecular pathways that underlie parathyroid development were discovered using pluripotent stem cells by Bingham and colleagues in 2009. These molecular pathways can be used to mimic parathyroid development in vitro and generation of cells for cellular replacement therapy. In vitro parathyroid regeneration was investigated using human embryonic stem cell (hESC) lines. BG01 cells were chosen as a model system because of their capacity to express several endoderm development markers during undirected differentiation into EBs, indicating that the cell line has not acquired abnormalities that would impair its ability to differentiate into endoderm. They optimized the expression of definitive markers of parathyroid development by modifying and extending existing techniques for the differentiation of mouse embryonic stem cells into definitive endoderm. The suitable technique was employing 100 ng/mL Activin A for 5 days with BG01 cells cultured on murine embryonic fibroblasts under circumstances of rising serum concentration. After 5 days in tissue culture, the cells were allowed to develop further without the addition of murine fibroblasts but with the presence of continuous Activin A. The differentiation protocol was known as Bingham protocol and cells were seeded onto mouse embryonic fibroblasts (MEFs) as feeder layer and treated with Activin A and SHH for a total of 26 days to obtain parathyroid-like cells (Fig. 1) (Bingham et al. 2009). Another study conducted by the same group found that another human embryonic stem cell line (H1) had comparable outcomes to their first findings with BG01 cells. According to the findings, differentiated cells exhibited parathyroid-related markers such as CasR, GCM2, and PTH and produced PTH hormone (Fig. 1) (Woods Ignatoski et al. 2010).
In a thesis study published in 2016, induced pluripotent stem cells (iPSCs) were reprogrammed from patients’ somatic cells and then differentiated into parathyroid progenitors for personalized cellular therapy. The advantages of the utilization of iPSCs were to diminish immune rejection in this study. Clustered regularly interspaced short palindromic repeats (CRISPR) and transcription activator-like effector nucleases (TALEN) gene-editing technologies were used to insert green fluorescent protein (GFP) reporter into GCM2, PTH, and CasR regulatory sequences to detect differentiation of iPSCs into parathyroid gland cells and progenitors. They hypothesized that iPSCs were directly differentiated through the endodermal lineage with main parathyroid cell characteristics such as PTH secretion in low calcium level, CaSR, and vitamin D receptor expression. Additionally, GATA3 (+/−) mouse model was used to observe the effect of treatment for hypoparathyroidism. The functional efficacy of hypoparathyroidism in the GATA3 (+/−) mouse is critical to aid the production of parathyroid progenitors for transplantation. This model holds promise as the basis for developing a patient-specific cell-based therapy for hypoparathyroidism (Fig. 1) (Sargent 2017).
Lawton and coworkers developed parathyroid hormone expressing cells from hES and hiPS cells in 2020. Definitive endoderm, anterior foregut endoderm, and pharyngeal endoderm differentiation were conducted at different time points of the protocol by using various differentiation medium cocktails. For definitive endoderm differentiation, Activin A, Wnt family member 3A (Wnt3a), PD0332991, and retinoic acid (RA) were added for 5 days. Between day 6 and 9, SB431542, Noggin, PD0332991, and inhibitor of Wnt productions-2 (IWP-2) were utilized for anterior foregut endoderm differentiation. Finally, in order to reach pharyngeal endoderm differentiation, LY364947, RA, FGF10, cyclopamine, BMP4, Noggin, and dexamethasone were used from day 10 to day 37. Each differentiation was confirmed with gene expression, immunofluorescence, flow cytometry, and PTH secretion analysis. This differentiation protocol allowed the proper treatment choices for patients through recognition of biological determinants of secretion and expression of PTH (Fig. 1) (Lawton et al. 2020).
5 Derivation of Parathyroid Cells from Various Cell Sources
In addition to pluripotent stem cells, various cell types including tonsil-derived mesenchymal stem cells (Park et al. 2015) and thymus epithelial cells (Ignatoski et al. 2011) have been used in the derivation of parathyroid-like cells, and these differentiation protocols were indicated in Table 1. Park et al. showed the differentiation of tonsil-derived mesenchymal stem cells into parathyroid-like cells. The differentiation was performed with Bingham protocol as described above. Briefly, Activin A and SHH combination was applied for 21 days (Bingham et al. 2009). Differentiated cells secreted PTH and also when these cells were injected into rats that were fed a calcium-free diet, PTH level was renovated, and the survival rate was increased. This study has shown that these differentiated cells were new cell sources for parathyroid cell function restoration and osteoporosis therapy in the future for the first time in the literature (Park et al. 2015).
Ignatoski and colleagues indicated the new cell source as thymus epithelial cells for the differentiation of parathyroid-like cells by Bingham protocol (Bingham et al. 2009). This differentiation process was established based on the thymus development process in which thymus and parathyroid glands are arised from the same primordium. The important points of this protocol were summarized as follows. Firstly, this study had used human tissue and had controlled calcium-regulated PTH secretion. Finally, there is no genetic manipulation of cells. Taken together, this study had a potential candidate for the replacement of parathyroid function of patients by in vitro trans-differentiated cells (Table 1) (Ignatoski et al. 2011).
In 2016, Zhao and his coworker stated that adipose-derived stem cells can be used as a proper cell source for differentiation into parathyroid-like cells to treat hypoparathyroidism with autologous cell therapy. The reason for choosing the adipose tissue was their immunomodulatory effect and differentiation potential. The hypothesis of this study was the differentiated cells expressed PTH-related markers and composed blood calcium level (Table 1) (Zhao and Luo 2016).
6 Preclinical and Clinical Applications of Parathyroid Tissue Engineering
In 2020, 200.000 patients suffered from hypoparathyroidism in the United States, Europe, Japan, and South Korea (New Survey on World Hypoparathyroidism 2021). Only in the United States at least 80.000 patients had hypoparathyroidism in 2020 (New Survey on World Hypoparathyroidism 2021). Pre-clinical and clinical studies about parathyroid tissue engineering were summarized in Table 2.
Gökyürek and her co-workers managed to isolate parathyroid cells from human parathyroid tissue and created hydrogel scaffolds via 3D bioprinter in 2020. Because of the spherical shape of the parathyroid gland and the polygonal shape of the chief cells, cylindrical architecture with honeycomb design was picked for the scaffold rather than cornered designs such as square. To further reduce cell leakage and promote cell adherence to the scaffold, a close-pored first layer of the scaffold was printed on the first layer of the scaffold and broader pores were printed on the remaining two layers. The parathyroid cells were grown on alginate scaffolds that were 3D printed and had structural and mechanical qualities that were similar to those of the natural tissue. The capabilities of these 3D printed tissues substitute to support cell survival and PTH secretion. As a result, it has been demonstrated that functional parathyroid gland substitutes can be produced using autologous cells and 3D printing to replace the natural parathyroid gland (Table 2) (Gökyürek 2020).
Allotransplantation of parathyroid was successfully done from a 32-year-old woman to a living donor. There were no complications for both donor and recipient. After allotransplantation, the calcium level in serum and PTH secretion increased progressively. This study is one of the examples for transplantation of the parathyroid gland from healthy donors properly (Table 2) (Agha et al. 2016).
In another study, for severe hypoparathyroidism, allotransplantation of macroencapsulated parathyroid is a better option than substitution drug therapy. A 27-year-old male with renal failure-induced parathyroid hyperplasia donated his parathyroid tissue for study. Macroencapsulation was designed with 157 μM thick from polyvinylidene difluoride artificial membrane. This encapsulated graft was injected into the lumen of the deep femoral artery. After this allotransplantation, calcium level and PTH secretion turned to normal levels. Also, grafts still had functionality after 3 months (Table 2) (Khryshchanovich and Ghoussein 2016).
Picariello and co-workers conducted the microencapsulation of human parathyroid cells with alginate-polylysine-alginate membranes. 45 patients with primary and secondary hyperparathyroidism had surgery to collect pathological parathyroid tissues. Following the microencapsulation of cells, there was evidence of long-term survival, cell proliferation, and PTH secretion in vitro. This study is a potential candidate for patients suffering from hypoparathyroidism (Table 2) (Picariello et al. 2001).
In 2015, Park and colleagues showed that novel mesenchymal stem cells generated from human tonsillar tissues (tonsil-derived mesenchymal stem cells TMSC) were differentiated into PTH-secreting cells. As reported previously, differentiated TMSC (dTMSC) was only able to restore parathyroid function in vivo when placed on a Matrigel scaffold. In 2018, Park et al. improved gelatin-hydroxyphenyl propionic acid (GH) hydrogels (GHH) instead of Matrigel scaffolds in order to embed their PTH-producing TMSC for successful transplantation and therapeutic effects. Continuous PTH release from TMSC-embedded GHH sustained stable serum Ca2+ levels in transplanted mice. Taken together, their findings indicate that a GHH-based system is a more promising and superior stem cell scaffold than other hydrogels which is a requirement for successful clinical applications (Table 2) (Park et al. 2018).
In addition to cell therapy-based approaches, PTH itself is used in the treatment of many conditions. There is another approach for directly utilizing PTH in clinical trials for the treatment of bone and skeletal-related diseases (Arthur and Gronthos 2020). A previously submitted research showed the effect of PTH on bone fractures and mineralization capacity in postmenopausal women with osteoporosis. PTH was injected in 1637 postmenopausal women subcutaneously at two different doses. According to results, PTH injection caused decrement in risk of bone fractures and increment in the bone mineral density of total body (Neer et al. 2001).
Another study had developed the cell-free scaffold model to regenerate bone defects. For the first time in the literature, local pulsatile PTH delivery system was developed for supporting bone regeneration by mimicking bone remodeling. The daily PTH delivery was realized in a cell-free biomimetic nanofibrous scaffold for 21 days. This study is a novel model for local bone defects based on the significant results of enhancing bone regeneration (Dang et al. 2017).
Additionally, Ishizuya et al. demonstrated that the PTH had affected the osteoblast differentiation in a dose and time-dependent manner. Isolated osteoblastic cells from newborn rat calvaria were treated with PTH at a different time interval for 48 h. According to results, 6 h administration of PTH caused the osteoblast differentiation mostly compared to other groups. To conclude, PTH treatment affects the osteoblast differentiation based on the exposure time (Ishizuya et al. 1997).
Generation of parathyroid cells from pluripotent stem cells and other cell types might be a source to produce PTH for clinical purposes. Production of the high amount of PTH in culture and further purification might be used in addition to cell therapy for therapeutic purposes.
7 Conclusion
Cell therapy is an emerging field for regenerative medicine in recent years. Stem cells, tissue-specific progenitors, stem cell-derived products, organoid systems, and tissue engineering applications are of great importance for clinical applications. Development of organ systems in controlled culture systems might be a solution for future organ replacement therapies. Parathyroid glands are small organs which could be a suitable target for cell and organ transfer therapies. Derivation of functional parathyroid cells and organ-like structures from appropriate cell sources are required to establish clinically relevant protocols for therapeutic applications. Pluripotent stem cells including embryonic stem cells and induced pluripotent stem cells, as remarkable cell sources with unlimited proliferation and differentiation potential might be used for parathyroid cell engineering. Derivation of induced pluripotent stem cells from adult cell sources enables the patient-specific cell therapy strategies. Even more crucially, because the donor cells and the tissues generated from iPSCs have identical genetic profiles, the immune system does not reject them (Taylor et al. 2011). ESCs, which are gold standard pluripotent stem cells, have limitless proliferation capacity and can transform into a variety of cell types (Zakrzewski et al. 2019). Understanding the molecular mechanisms of pluripotent stem cell fate decision might allow derivation of clinically relevant cell sources for regenerative medicine.
Investigation of culture and differentiation conditions might enable obtaining fully functional parathyroid cells, parathyroid organoids, and establishment of organ-like structures in scaffold systems. Because parathyroid is a small endocrine organ, transplantation of in vitro derived cells, organoids, and tissue engineering constructs might be a prospective solution.
In this chapter, we summarized the current literature for parathyroid cell differentiation from various sources which might help researchers and clinicians in the near future.
Abbreviations
- BMP4:
-
Bone morphogenetic protein 4
- CaSR:
-
Calcium-sensitive receptor
- CCL21:
-
C-C motif chemokine ligand 21
- CRISPR:
-
Clustered regularly interspaced short palindromic repeats
- dTMSC:
-
Differentiated TMSC
- EBs:
-
Embryoid bodies
- ECF:
-
Extracellular fluid
- EYA1:
-
EYA transcriptional coactivator and phosphatase 1
- FGF23:
-
Fibroblast growth factor 23
- FOXN1:
-
Forkhead box N1
- GATA3:
-
GATA binding protein 3
- GCM2:
-
Glial cells missing transcription factor 2
- GFP:
-
Green fluorescent protein
- GHH:
-
Gelatin-hydroxyphenyl propionic acid (GH) hydrogels
- hESC:
-
Human embryonic stem cell
- HOXA3:
-
Homeobox A3
- iPSCs:
-
Induced pluripotent stem cells
- IWP-2:
-
Inhibitor of Wnt productions-2
- MAFB:
-
MAF BZIP transcription factor B
- MEFs:
-
Mouse embryonic fibroblasts
- PAX1:
-
Paired box 1
- PAX9:
-
Paired box 9
- PKA:
-
Protein kinase A
- PTH:
-
Parathyroid hormone
- PTH1R:
-
Type 1 parathyroid hormone receptor
- PTH2R:
-
Type 2 parathyroid hormone receptor
- PTHrP:
-
Parathyroid hormone-related protein
- RA:
-
Retinoic acid
- SHH:
-
Sonic hedgehog
- TALEN:
-
Transcription activator-like effector nucleases
- TBX1:
-
T-box transcription factor
- TGFs:
-
Transforming growth factors
- TMSC:
-
Tonsil-derived mesenchymal stem cells
- Wnt3a:
-
Wnt family member 3A
References
Agha A, Scherer MN, Moser C et al (2016) Living-donor parathyroid allotransplantation for therapy-refractory postsurgical persistent hypoparathyroidism in a nontransplant recipient – three year results: a case report. BMC Surg 16. https://doi.org/10.1186/s12893-016-0165-y
Arthur A, Gronthos S (2020) Clinical application of bone marrow mesenchymal stem/stromal cells to repair skeletal tissue. Int J Mol Sci 21:1–27
Bellido T, Saini V, Pajevic PD (2013) Effects of PTH on osteocyte function. Bone 54:250–257. https://doi.org/10.1016/j.bone.2012.09.016
Bingham EL, Cheng SP, Woods Ignatoski KM, Doherty GM (2009) Differentiation of human embryonic stem cells to a parathyroid-like phenotype. Stem Cells Dev 18:1071–1080. https://doi.org/10.1089/scd.2008.0337
Bleul CC, Boehm T (2005) BMP signaling is required for normal thymus development. J Immunol 175:5213–5221. https://doi.org/10.4049/JIMMUNOL.175.8.5213
Bro S, Olgaard K (1997) Effects of excess PTH on nonclassical target organs. Am J Kidney Dis 30:606–620
Brown MB, Limaiem F (2020) Histology. StatPearls Publishing, Parathyroid Gland
Canaff L, Zhou X, Hendy GN (2008) The Proinflammatory cytokine, Interleukin-6, up-regulates calcium-sensing receptor gene transcription via Stat1/3 and Sp1/3 *. J Biol Chem 283:13586–13600. https://doi.org/10.1074/JBC.M708087200
Casale J, Giwa AO (2019) Embryology, branchial arches. StatPearls Publishing
Chambers TJ, Fuller K, McSheehy PMJ, Pringle JAS (1985) The effects of calcium regulating hormones on bone resorption by isolated human osteoclastoma cells. J Pathol 145:297–305. https://doi.org/10.1002/path.1711450403
Cipriani NA (2014) Parathyroid: pathophysiology and molecular mechanisms of disease. In: Pathobiology of human disease: a dynamic encyclopedia of disease mechanisms. Elsevier, pp 1234–1241
Clemens TL, Cormier S, Eichinger A et al (2001) Parathyroid hormone-related protein and its receptors: nuclear functions and roles in the renal and cardiovascular systems, the placental trophoblasts and the pancreatic islets. Br J Pharmacol 134:1113–1136
Cordier AC, Haumont SM (1980) Development of thymus, parathyroids, and ultimo-branchial bodies in NMRI and nude mice. Am J Anat 157:227–263. https://doi.org/10.1002/aja.1001570303
Dang M, Koh AJ, Jin X et al (2017) Local pulsatile PTH delivery regenerates bone defects via enhanced bone remodeling in a cell-free scaffold. Biomaterials 114:1–9. https://doi.org/10.1016/j.biomaterials.2016.10.049
Gardiner JR, Jackson AL, Gordon J et al (2012) Localised inhibition of FGF signalling in the third pharyngeal pouch is required for normal thymus and parathyroid organogenesis. Development 139:3456–3466. https://doi.org/10.1242/dev.079400
Gilmour JR (1937) The embryology of the parathyroid glands, the thymus and certain associated rudiments. J Pathol Bacteriol 45:507–522. https://doi.org/10.1002/path.1700450304
Gökyürek M (2020) Ankara University graduate school of natural and applied sciences master of science thesis 3D bioprinting of parathyroid tissue. Ankara: Ankara Üniversitesi: Fen Bilimleri Enstitüsü: Mühendislik Fakültesi: Biyomedikal Mühendisliği Ana Bilim Dalı
Gordon J, Bennett AR, Blackburn CC, Manley NR (2001) Gcm2 and Foxn1 mark early parathyroid- and thymus-specific domains in the developing third pharyngeal pouch. Mech Dev 103:141–143. https://doi.org/10.1016/S0925-4773(01)00333-1
Gordon J, Patel SR, Mishina Y, Manley NR (2010) Evidence for an early role for BMP4 signaling in thymus and parathyroid morphogenesis. Dev Biol 339:141–154. https://doi.org/10.1016/j.ydbio.2009.12.026
Greenspan SL, Bone HG, Ettinger MP et al (2007) Effect of recombinant human parathyroid hormone (1-84) on vertebral fracture and bone mineral density in postmenopausal women with osteoporosis. A randomized trial. Ann Intern Med 146:326–339. https://doi.org/10.7326/0003-4819-146-5-200703060-00005
Günther T, Chen Z-F, Kim J et al (2000) Genetic ablation of parathyroid glands reveals another source of parathyroid hormone. Nature 406:199–203. https://doi.org/10.1038/35018111
Hosoya T, Takizawa K, Nitta K, Hotta Y (1995) Glial cells missing: a binary switch between neuronal and glial determination in drosophila. Cell 82:1025–1036. https://doi.org/10.1016/0092-8674(95)90281-3
Ignatoski KMW, Bingham EL, Frome LK, Doherty GM (2011) Directed trans-differentiation of thymus cells into parathyroid-like cells without genetic manipulation. Tissue Eng – Part C Methods 17:1051–1059. https://doi.org/10.1089/ten.tec.2011.0170
Ishizuya T, Yokose S, Hori M et al (1997) Parathyroid hormone exerts disparate effects on osteoblast differentiation depending on exposure time in rat osteoblastic cells. J Clin Invest 99:2961–2970. https://doi.org/10.1172/JCI119491
Iwaniec UT, Moore K, Rivera MF et al (2007) A comparative study of the bone-restorative efficacy of anabolic agents in aged ovariectomized rats. Osteoporos Int 18:351–362. https://doi.org/10.1007/s00198-006-0240-9
Jayakumar A (2012) Greenspan’s basic and clinical endocrinology. Yale J Biol Med 85:559
Khan M, Sharma S (2018) Physiology, parathyroid hormone (PTH). StatPearls Publishing
Khryshchanovich V, Ghoussein Y (2016) Allotransplantation of macroencapsulated parathyroid cells as a treatment of severe postsurgical hypoparathyroidism: case report. Ann Saudi Med 36:143–147. https://doi.org/10.5144/0256-4947.2016.21.3.1130
Kim J, Jones BW, Zock C et al (1998) Isolation and characterization of mammalian homologs of the Drosophila gene glial cells missing. Proc Natl Acad Sci U S A 95:12364–12369. https://doi.org/10.1073/pnas.95.21.12364
Lawton BR, Martineau C, Sosa JA et al (2020) Differentiation of PTH-expressing cells from human pluripotent stem cells. Endocrinology 161. https://doi.org/10.1210/endocr/bqaa141
Liu C, Saito F, Liu Z et al (2006) Coordination between CCR7- and CCR9-mediated chemokine signals in prevascular fetal thymus colonization. Blood 108:2531–2539. https://doi.org/10.1182/blood-2006-05-024190
Liu Z, Yu S, Manley NR (2007) Gcm2 is required for the differentiation and survival of parathyroid precursor cells in the parathyroid/thymus primordia. Dev Biol 305:333–346. https://doi.org/10.1016/j.ydbio.2007.02.014
Liu Z, Farley A, Chen L et al (2010) Thymus-associated parathyroid hormone has two cellular origins with distinct endocrine and immunological functions. PLoS Genet 6:e1001251. https://doi.org/10.1371/journal.pgen.1001251
Mannstadt M, Jüppner H, Gardella TJ (1999) Receptors for PTH and PTHrP: their biological importance and functional properties. Am J Physiol – Ren Physiol 277:F665–F675
Marx SJ (2000) Hyperparathyroid and hypoparathyroid disorders. N Engl J Med 343:1863–1875. https://doi.org/10.1056/nejm200012213432508
Mason JM, Morrison DJ, Basson MA, Licht JD (2006) Sprouty proteins: multifaceted negative-feedback regulators of receptor tyrosine kinase signaling. Trends Cell Biol 16:45–54. https://doi.org/10.1016/J.TCB.2005.11.004
McSheehy PMJ, Chambers TJ (1986) Osteoblastic cells mediate osteoclastic responsiveness to parathyroid hormone. Endocrinology 118:824–828. https://doi.org/10.1210/endo-118-2-824
Mizobuchi M, Ritter CS, Krits I et al (2009) Calcium-sensing receptor expression is regulated by glial cells missing-2 in human parathyroid cells. J Bone Miner Res 24:1173–1179. https://doi.org/10.1359/JBMR.090211
Neer RM, Arnaud CD, Zanchetta JR et al (2001) Effect of parathyroid hormone (1-34) on fractures and Bone mineral density in postmenopausal women with osteoporosis. N Engl J Med 344:1434–1441. https://doi.org/10.1056/nejm200105103441904
Neubueser A, Koseki H, Balling R (1995) Characterization and developmental expression of Pax9, a paired-box-containing gene related to Pax1. Dev Biol 170:701–716. https://doi.org/10.1006/DBIO.1995.1248
New Survey on World Hypoparathyroidism Day Highlights Need for Awareness of this Rare Endocrine Disorder and Patient Challenges to Receive Adequate Care. https://www.globenewswire.com/news-release/2020/06/01/2041595/0/en/New-Survey-on-World-Hypoparathyroidism-Day-Highlights-Need-for-Awareness-of-this-Rare-Endocrine-Disorder-and-Patient-Challenges-to-Receive-Adequate-Care.html. Accessed 25 Apr 2021
Okabe M, Graham A (2004) The origin of the parathyroid gland. Proc Natl Acad Sci 101:17716–17719. https://doi.org/10.1073/PNAS.0406116101
Park YS, Kim HS, Jin YM et al (2015) Differentiated tonsil-derived mesenchymal stem cells embedded in Matrigel restore parathyroid cell functions in rats with parathyroidectomy. Biomaterials 65:140–152. https://doi.org/10.1016/j.biomaterials.2015.06.044
Park YS, Lee Y, Jin YM et al (2018) Sustained release of parathyroid hormone via in situ cross-linking gelatin hydrogels improves the therapeutic potential of tonsil-derived mesenchymal stem cells for hypoparathyroidism. J Tissue Eng Regen Med 12:e1747–e1756. https://doi.org/10.1002/term.2430
Peters H, Neubüser A, Kratochwil K, Balling R (1998) Pax9-deficient mice lack pharyngeal pouch derivatives and teeth and exhibit craniofacial and limb abnormalities. Genes Dev 12:2735–2747. https://doi.org/10.1101/GAD.12.17.2735
Pettway GJ, Schneider A, Koh AJ et al (2005) Anabolic actions of PTH (1-34): use of a novel tissue engineering model to investigate temporal effects on bone. Bone 36:959–970. https://doi.org/10.1016/j.bone.2005.02.015
Picariello L, Benvenuti S, Recenti R et al (2001) Microencapsulation of human parathyroid cells: an “in vitro” study. J Surg Res 96:81–89. https://doi.org/10.1006/jsre.2000.6054
Renkema KY, Alexander RT, Bindels RJ, Hoenderop JG (2008) Calcium and phosphate homeostasis: concerted interplay of new regulators. Ann Med 40:82–91
Rosen RD, Bordoni B (2020) Embryology, parathyroid. StatPearls Publishing
Sargent RG (2017) Derivation of parathyroid gland cells and their progenitors from induced Pluripotent Stem Cells (iPSCs) for personalized therapy. Technical report 2017. University of California, San Francisco. https://apps.dtic.mil/sti/citations/AD1108198
Sato E, Muto J, Zhang L-J et al (2016) The parathyroid hormone second receptor PTH2R and its ligand Tuberoinfundibular peptide of 39 residues TIP39 regulate intracellular calcium and influence keratinocyte differentiation. J Invest Dermatol 136:1449–1459. https://doi.org/10.1016/J.JID.2016.02.814
Shaker JL, Deftos L (2013) Calcium and phosphate homeostasis. In: Endocrine and reproductive physiology. Elsevier, pp 77–e1
Shandiz AE, Lee A, Shingo Suzuki RG, Sargent MH, Yezzi LA, Orloff DCG (2013) 472. Directed differentiation of human induced pluripotent stem (hiPS) cells into functional parathyroid tissue for cell therapy. Mol Ther 21:S181–S182. https://doi.org/10.1016/s1525-0016(16)34807-9
Silva BC, Costa AG, Cusano NE et al (2011) Catabolic and anabolic actions of parathyroid hormone on the skeleton. J Endocrinol Investig 34:801–810
Strewler GJ (2009) The physiology of parathyroid hormone–related protein. N Engl J Med 342:177–185. https://doi.org/10.1056/NEJM200001203420306
Su D -m, Ellis S, Napier A et al (2001) Hoxa3 and Pax1 regulate epithelial cell death and proliferation during thymus and parathyroid organogenesis. Dev Biol 236:316–329. https://doi.org/10.1006/DBIO.2001.0342
Talmage RV, Mobley HT (2008) Calcium homeostasis: reassessment of the actions of parathyroid hormone. Gen Comp Endocrinol 156:1–8
Tanaka Y, Funahashi H, Imai T et al (1996) Oxyphil cell function in secondary parathyroid hyperplasia? Nephron 73:580–586. https://doi.org/10.1159/000189144
Taterra D, Wong LM, Vikse J et al (2019) The prevalence and anatomy of parathyroid glands: a meta-analysis with implications for parathyroid surgery. Langenbeck’s Arch Surg 404:63–70. https://doi.org/10.1007/s00423-019-01751-8
Taylor CJ, Bolton EM, Bradley JA (2011) Immunological considerations for embryonic and induced pluripotent stem cell banking. Philos Trans R Soc B Biol Sci 366:2312. https://doi.org/10.1098/RSTB.2011.0030
Woods Ignatoski KM, Bingham EL, Frome LK, Doherty GM (2010) Differentiation of precursors into parathyroid-like cells for treatment of hypoparathyroidism. Surgery 148:1186–1190. https://doi.org/10.1016/j.surg.2010.09.021
Xu P-X, Zheng W, Laclef C et al (2002) Eya1 is required for the morphogenesis of mammalian thymus, parathyroid and thyroid. Development 129:3033–3044. https://doi.org/10.1242/DEV.129.13.3033
Yamada T, Tatsumi N, Anraku A et al (2019) Gcm2 regulates the maintenance of parathyroid cells in adult mice. PLoS One 14:e0210662. https://doi.org/10.1371/JOURNAL.PONE.0210662
Yang D, Singh R, Divieti P et al (2007) Contributions of parathyroid hormone (PTH)/PTH-related peptide receptor signaling pathways to the anabolic effect of PTH on bone. Bone 40:1453–1461. https://doi.org/10.1016/j.bone.2007.02.001
Zakrzewski W, Dobrzyński M, Szymonowicz M, Rybak Z (2019) Stem cells: past, present, and future. Stem Cell Res Ther 10. https://doi.org/10.1186/S13287-019-1165-5
Zhao Y, Luo B (2016) Adipose-derived stem cells: a novel source of parathyroid cells for treatment of hypoparathyroidism. Med Hypotheses 93:143–145. https://doi.org/10.1016/j.mehy.2016.05.011
Acknowledgments
This study was supported by TÜBİTAK-ARDEB 1001 Program (Project no: 219S665). The authors declare no conflict of interest.
Author information
Authors and Affiliations
Corresponding author
Editor information
Editors and Affiliations
Rights and permissions
Copyright information
© 2021 Springer Nature Switzerland AG
About this chapter
Cite this chapter
Şenkal, S., Doğan, A. (2021). Parathyroid Cell Differentiation from Progenitor Cells and Stem Cells: Development, Molecular Mechanism, Function, and Tissue Engineering. In: Turksen, K. (eds) Cell Biology and Translational Medicine, Volume 16. Advances in Experimental Medicine and Biology(), vol 1387. Springer, Cham. https://doi.org/10.1007/5584_2021_694
Download citation
DOI: https://doi.org/10.1007/5584_2021_694
Published:
Publisher Name: Springer, Cham
Print ISBN: 978-3-031-10637-8
Online ISBN: 978-3-031-10638-5
eBook Packages: Biomedical and Life SciencesBiomedical and Life Sciences (R0)